Effects of unidirectional flow shear stresses on the formation, fractal microstructure and rigidity of incipient whole blood clots and fibrin gels
Abstract
Incipient clot formation in whole blood and fibrin gels was studied by the rheometric techniques of controlled stress parallel superposition (CSPS) and small amplitude oscillatory shear (SAOS). The effects of unidirectional shear stress on incipient clot microstructure, formation kinetics and elasticity are reported in terms of the fractal dimension (df) of the fibrin network, the gel network formation time (TGP) and the shear elastic modulus, respectively. The results of this first haemorheological application of CSPS reveal the marked sensitivity of incipient clot microstructure to physiologically relevant levels of shear stress, these being an order of magnitude lower than have previously been studied by SAOS. CSPS tests revealed that exposure of forming clots to increasing levels of shear stress produces a corresponding elevation in df, consistent with the formation of tighter, more compact clot microstructures under unidirectional flow. A corresponding increase in shear elasticity was recorded. The scaling relationship established between shear elasticity and df for fibrin clots and whole blood confirms the fibrin network as the dominant microstructural component of the incipient clot in terms of its response to imposed stress. Supplementary studies of fibrin clot formation by rheometry and microscopy revealed the substantial additional network mass required to increase df and provide evidence to support the hypothesis that microstructural changes in blood clotted under unidirectional shear may be attributed to flow enhanced thrombin generation and activation. CSPS also identified a threshold value of unidirectional shear stress above which no incipient clot formation could be detected. CSPS was shown to be a valuable haemorheological tool for the study of the effects of physiological and pathological levels of shear on clot properties.
1Introduction
Blood coagulation involves the formation of haemostatic plugs called clots at lesions of the circulatory system. In addition to the initial haemostatic response of platelets to subendothelial cells or foreignsurfaces [20, 31], the clotting process involves fibrinogen-fibrin transformation due to the catalytic action of thrombin and the subsequent polymerisation and network formation of fibrin fibres which serves to stabilise the initial platelet plug [1, 38]. In vivo the network of fibrin fibres forms the primary microstructural basis of a clot whereas in vitro they form a fibrin gel [26].
Viscoelastic properties are among the most sensitive measures of fibrin polymerization and clot microstructure [38]. In haemorheological measurements the clotting time may be identified with that required to form a sample-spanning network of fibrin fibres at the Gel Point, GP [13, 39]. The GP marks the viscoelastic fluid to solid transition and corresponds to the establishment of the incipient clot [14]. Accurate techniques for GP detection are based on measurements of the viscoelastic properties of incipient clots [39]. A fractal analysis of such measurements quantifies incipient clot microstructure in fibrin gels and blood in terms of a fractal dimension, df [12, 14, 15, 22, 23]. In healthy whole blood a narrow range of df (1.74±0.04) provides a Healthy Index for normal clotting [14]. Enhanced clot rigidity, higher internal connectivity of fibrin network mass and decreased permeability correspond to higher values of df whereas lower values, such as those reported in anticoagulated blood, correspond to weaker gels with more open, less dense fibrin networks [12, 14, 15].
The ability to quantify clot microstructure has clinical significance. In vitro clot microstructure correlates with epidemiological and clinical data in cardiovascular diseases [36]. Patients with thrombotic disorders form plasma clots in vitro with altered microstructure, these clots being more rigid and less permeable than those from control subjects [24]. Among the many factors that affect fibrin clot microstructure is shear flow, which modulates the kinetics of fibrin formation and polymerisation [29]. Flow has a direct effect on individual platelets, including exposure of anionic phospholipids, which provide a surface for thrombin generation [25]. The shear rates associated with physiological flows lead to activation of platelets and thrombin release, with high levels of thrombin resulting in the production of thinner fibrin fibres with greater number of branchpoints [39]. Although flow can wash away thrombin or agonists released from platelets like ADP and hence decrease clotting, it can also result in a ‘denser’ microstructure by bringing more fibrinogen to the forming clot [29]. Fibrin fibre orientation along the direction of flow [3, 17, 41] also has important consequences for clot mechanical properties and susceptibility to fibrinolysis [3, 37] and it has been proposed that shear forces of the blood stream determine the likelihood of embolization [7].
The effects of flow on the microstructure of blood clots at the earliest (incipient) stage of their formation have not previously been reported. This is a significant omission insofar as the incipient fibrin clot provides a microstructural template for ensuing clot development [12]. Flow induced changes in the assembly of the template may thus have significant consequences for clot elasticity and susceptibility to fibrinolysis and hence for clot quality [3]. The present study was undertaken to elucidate the consequences of imposing unidirectional flow shear stresses on the microstructural and mechanical properties of incipient clots, as they formed, in whole blood and fibrin gels. The rheometric technique employed was controlled stress parallel superposition, CSPS, the present work being the first to employ CSPS to study blood clot formation. Additional rheometric and microscopy studies were undertaken on samples of blood and fibrin clots.
2Experimental
2.1Small amplitude oscillatory shear, SAOS
A controlled stress rheometer (AR-G2, TA Instruments) was used to measure the elastic and viscous components (G′ and G′′, respectively) of the complex shear modulus, G*. At the GP, G′ and G′′ scale in oscillatory frequency, ω, as G′(ω) ∼ G′′(ω) ∼ ωα [5]. Thus the GP is identified by attainment of frequency independence of the loss tangent, tanδ, where tanδ= G′′/G′ and δ is the phase angle between oscillatory stress and strain waveforms.
The microstructure of dilute fibrin gels, at relatively low fibrinogen concentration, has previously been reported in terms of a fractal analysis of light scattering data [16]. For more concentrated systems such as whole blood a fractal analysis of viscoelastic GP data provides a basis for quantifying the structural complexity of the incipient clot’s fibrin network [12, 14, 15, 22, 23]. Prior to the GP isolated clusters of branching fibrin fibres formed from polymerized monomers grow in the sol phase as loosely coupled bundles of flexible polymers [30]. At the GP a sample-spanning gel network is established as a continuous path between the sample’s bounding surfaces. The polymerizing system is macroscopically homogeneous at a length-scale L greater than the correlation length ɛ, whereas for L ⪡ ɛ the network cluster is a fractal object whose mass M scales as M ∼ Ldf, where df is the fractal dimension of the network. The greater the value of df the more compact is the network, whereas lower values of df correspond to more open/permeable networks. The exponent α obtained from GP measurements is a measure of gel network branching and is related to df as df= (D + 2)(2α – D)/2(α – D), where D is the Euclidian dimension (D = 3) [28]. It is important to note that df is inferred from the power law scaling in both the storage (elastic) and viscous (loss) moduli at the gel point, the scaling exponent α (and thus df) being independent of the values of the storage modulus, G′, at any single frequency.
In fibrin-thrombin gels formed over a range of thrombin concentration, incipient clots are characterised by values of df consistent with limiting values for fractal clusters formed by diffusion limited cluster–cluster aggregation and reaction limited cluster–cluster aggregation processes [11, 33, 40].
2.2Controlled stress parallel superposition, CSPS
CSPS was used to apply combined unidirectional and oscillatory shear stresses (σs and σo, respectively) to samples of clotting blood and fibrin-thrombin solutions, the resulting unidirectional and oscillatory flow components being parallel to each other (see Fig. 1). It is interesting to note that a similar, though significantly more complex, superposed flow regime exists within the pulsatile flow dynamics of the circulatory system. The net accumulated strain is zero under SAOS whereas the accumulated unidirectional strain increases progressively in CSPS. In controlled deformation rate superposition, the flow is described in terms of its oscillatory and steady components. This is not appropriate to the present CSPS work, which involves a time-varying unidirectional shear rate under constant stress due to rheological changes during clotting. Thus we refer herein to the unidirectional flow component, rather than a steady component.
The shear stress in parallel superposition at low amplitude is given by:
(1)
(2)
(3)
In the present experiments,
2.3Blood samples
Blood was collected from 8 healthy volunteers following informed consent and full ethical approval. All donors denied taking any medication for two weeks prior to the day of collection, on which blood was withdrawn into 5–7 Vacutainertrademark tubes (4.5 mL; Becton & Dickinson Co., UK) containing 3.8% sodium citrate to form a mixture of 9:1 vol./vol. blood to citrate, respectively. This was done, without any stasis, from an antecubital vein by venipuncture using a 21-gauge butterfly needle (Greiner Bio-one GmbH–Austria). Sodium citrate, an anticoagulant that binds the calcium ions in the blood, prevented further coagulation. Its effect was reversed by adding 0.2 M Calcium Chloride, CaCl2 (Sigma-Aldrich) [42] to a final concentration of 0.005 M. The citrated blood was kept in a water bath at 37°C during the experiment and was used, in every case, within 4 hours of collection.
SAOS and CSPS measurements were performed using an aluminium parallel plate measuring geometry (6 cm diameter). In this geometry, unlike the cone and plate, the shear rate is not uniform throughout the shearing gap. However, the presence of cellular material within whole blood samples precluded the use of the latter. Samples of blood were loaded by pipette onto the temperature controlled lower plate (37°C), the upper plate being immediately lowered thereafter to the preset shearing gap (300 μm). A thin layer of low viscosity (9.4 mPa.s) silicon oil prevented evaporation. The peak oscillatory stress, σ0, was set at a level which ensured adequate waveform resolution and linear viscoelastic responses. The steady applied stress σs used to generate the unidirectional flow was adjusted in each experiment.
2.4Fibrin-thrombin gels
Human fibrinogen (43.0 mg/ml) and human-α-thrombin (500 NIH/ml) were obtained from Enzyme Research Laboratories Ltd, UK, and prepared as instructed by the manufacturer. Aliquots were stored at −80°C until required. Samples of human albumin (Sigma Aldrich, 10 wt% ), and CaCl2 (Fluka, 1 M) were stored at 4°C until required. Samples of fibrinogen and thrombin were allowed to thaw at room temperature before being placed on ice. Appropriate amounts of albumin diluted to 4.5 wt% in Tris Buffered Saline (Sigma Aldrich), fibrinogen and CaCl2 were mixed (in that order) to give the required final concentration of c= 10 mg/ml fibrinogen and 0.005 M CaCl2. Thrombin was added to initiate gelation at a final concentration φ= 0.07 NIH/ml immediately prior to the sample’s transfer to a custom made lower plate mounted on the temperature-controlled stage of the rheometer.
The plate incorporated a glass cover slip providing an optical path through the sample. The stage and plate temperature was maintained at 10°C. A thin (∼10 μm) polyethylene film secured on the upper Cone (60 mm diameter, 2° cone angle) provided a defect free, readily removed cover over the surface of the rheometer measuring geometry. The sample’s outer free surface was covered with a thin layer of low viscosity silicon oil to minimise evaporative losses. A series of CSPS measurements were conducted on fibrinogen-thrombin gels (all c = 10 mg/ml, φ= 0.07 NIH/ml) and the steady applied stress σs used to generate the unidirectional flow was adjusted in each experiment.
2.5Laser Scanning Confocal Microscopy, LSCM
Following the attainment of a GP in the experiments described in Section 2.4, the rheometer geometry was locked in position and a scalpel was used to release and fold back the polyethylene film. The geometry was subsequently raised to the loading position, leaving the fibrin gel covered by the film. The aluminium plate was immediately mounted on the stage of a Zeiss LSM 710 inverted confocal microscope. A 63× (1.4 N.A.) oil immersion objective was focused through the cover slip built into the aluminium plate to a distance of approximately 20 μm into the fibrin gel. Using a 488 nm Argon ion laser and the microscope set in reflection mode, a stack of images measuring 135 μm (W) x 135 μm (H) x 40 μm (D) was recorded.
2.6Scanning Electron Microscopy, SEM
After recording LSCM images, the same sample used in CSPS and LSCM was transferred to a Petri dish and washed three times with 50 mM Sodium Cacodylate-HCl Buffer solution (pH 7.2–7.4, SPI Supplies) at 10 to 20 minute intervals to remove excess salt. The gel was fixed overnight in 2% Glutaraldehyde (Sigma Aldrich, UK) and the sample was dehydrated with a series of graded concentrations (30% to 100 % ) of ethanol. The dehydrated sample was then rinsed with 50% Hexamethyldisilazane solution (HMDS) in 100% ethanol for 10 minutes in a fume hood and then three times in 100% HMDS and left overnight to dry. The sample was coated with a thin layer of gold (∼15 nm) using sputter coating and was imaged using scanning electron microscopy (Hitachi 4800).
3Results
For incipient blood clots the value of df (1.75) in SAOS (σs= 0 Pa) is within the Healthy Index range previously reported [14]. As σs is increased progressively in a series of CSPS tests, the value of df increases from df= 1.75 to a maximum value (df= 2.20) at σs= 0.141 Pa. Further increases in σs, to 0.235 Pa, produced no further significant increase in df and no evidence of incipient clot formation could be detected (no GP was recorded) for σs > 0.235Pa. The values of the incipient clot formation time, TGP, under CSPS increased from 133 (±37) seconds (at σs= 0 Pa) to 182 (±43) seconds at σs= 0.141 Pa. No further increase in TGP was recorded for stresses in the range 0.141 < σs < 0.235Pa (see Fig. 2). An example of the CSPS results obtained for blood is shown in Fig. 3 whilst Fig. 4 shows the power law scaling of G′ and G′′ for a fibrin thrombin gel under SAOS.
Figure 5 shows the relationship between df and levels of incipient clot elasticity,
The LSCM images shown in Fig. 6 were recorded immediately following attainment of the GP and illustrate the striking effects of unidirectional shear flow on fibrin clot microstructure. The fibrin network structure incorporates progressively more mass as the unidirectional flow stress σs increases, a finding consistent with the elevated values of df found in CSPS.
The scanning electron micrographs (SEMs) (see Fig. 7) obtained for the same samples used in confocal imaging and rheology tests reveal that, the distribution of fibrin fibre diameter increases as the shear stress increases. The fibre diameter distribution (see Fig. 8) was analysed using Image J (V 510, National Institutes of Health) by placing a random grid of crosses (500 crosses/image) on images and using the line tool to measure fibre diameter. Networks formed at σs= 0 Pa (i.e. under SAOS) exhibited the characteristic homogenous/quiescent gel. In contrast, networks formed under different levels of superposed shear stress (σs= 0.1, 0.17, 0.35 Pa) showed a prominent network of thick fibres, as well as fibre ‘aggregates’ in which multiple individual fibres were coalesced into bundles, several of which had diameters greater than 1 μm. These changes could be the result of physical forces of stress on the fibre growth or the transport of oligomers as a result of the stress.
4Discussion
The unidirectional shear stresses employed in this CSPS study are lower than those previously reported to influence the elasticity of fully formed clots in SAOS tests following exposure of coagulating plasma to flow [18]. A significant aspect of the CSPS results is that they reveal the marked sensitivity of incipient blood clot microstructure (df) to unidirectional flow shear stresses <0.3 Pa. Such low stresses havephysiological relevance insofar as they are commensurate with estimates of the mean wall shear stress for flow in the aorta, inferior vena cava and other venous structures [6, 21]. The potential clinical significance of the increase in df under CSPS is that fractal networks such as the incipient clot require large amounts of additional mass to produce small increments in df. Further, given the incipient clot’s role as a microstructural template for ensuing clot development [12], elevated values of df might lead to the establishment of denser, less permeable clots with enhanced resistance to fibrinolysis [8–10] and deformation [32]. The latter characteristic is commensurate with the present results in terms of the significant correlation between df and shear elastic modulus,
A striking finding is that all the results for df and normalized elasticity for blood (under CSPS) and fibrin gels (under SAOS and CSPS) are represented by a single exponential relationship (see Fig. 5) – a feature consistent with the underlying fractal nature of the fibrin network assembly. Despite the presence of cellular material in whole blood, the relationship between clot microstructure (df) and elasticity is the same as that found in fibrin clots – systems which have no other microstructural component. Thus, in terms of this ‘structure-function’ relationship, the present results confirm the fibrin network as the dominant microstructural component of the incipient clot in terms of determining its response to imposed stress.
The incorporation of greater mass within the fibrin network under flow would be expected to result in increased elasticity as the shear elastic modulus correlates with branchpoint density and elevated values of the latter correspond to higher values of df [32]. High levels of thrombin are associated with the production of thin fibrin fibres with many branchpoints and enhanced monomer activation being expected to result from more effective mixing of the available thrombin under flow [1, 26]. Molecular dynamic simulations (MDS) of incipient fractal clot formation, based on the activation-limited aggregation of rod-like particles into sample-spanning GP clusters, reveal that enhanced monomer activation, generated by activation profiles representing differing rates of thrombin production, increases (i) the rate of addition of mass inside the growing molecular clusters; and (ii) the diffusion and transport of mass in the intra-cluster voids [11]. Hence, under the enhanced monomer activation resulting from mixing in shear flow, clots form from smaller units with relatively large amounts of incorporated mass, and correspondingly high df. This suggests that the increase in df in blood may be attributable to shear enhanced thrombin generation.
A previous SAOS study of fibrin gel formation reported that at high (ab initio) thrombin concentration df reached a limiting maximum value (ca. df ∼ 2.0) [12]. Under CSPS, at the same values of c and φ, the value of df increases progressively in the range 2.0 < df < 2.3 with increasing stress σs. These findings indicate that in the absence of the mixing/activation effect of a unidirectional shear flow, ab initio thrombin addition cannot alone induce sufficient activation to produce the substantial additional fibrin mass required to achieve the highest values of df (>2.0) recorded in CSPS.
Following an initial increase in incipient clot formation time TGP in coagulating blood under CSPS at low stresses, no further increase was observed in the range 0.141 < σs < 0.235 Pa. This may be understood in terms of the disruptive influence of the unidirectional flow on the growing pre-GP clusters as the accumulated strain increases during clotting. Carvalho and Djabourov [4] have reported biopolymer gelation under alternating sequences of oscillatory flow and steady shear flow in which the flow kinematics caused gel disruption. In the present CSPS work on blood, the unidirectional flow produces two competing effects. While the shear field serves to delay the GP, enhanced platelet activation would eventually counterbalance this effect to produce the constant value of TGP and result in a higher value of df when the incipient clot eventually forms (i.e. when the competing effect of pre-GP cluster growth is sufficient to establish a sample-spanning network). The maximum unidirectional shear rates in the CSPS experiments were typically less than 10 s −1, the increase in df recorded under these conditions being consistent with observations by Campbell et al. [3] who note that shear flow modulates the kinetics of both fibrin monomer formation and polymerisation. Ultimately, at sufficiently high stress, no GP is recorded under CSPS, a finding commensurate with a reported decrease in platelet-fibrin interaction with increasing shear stress exposure at sufficiently high levels of stress in SAOS studies [18]. Given that there is no (permanent) fibrin fibre orientation under SAOS, it is interesting to consider whether the elevated values of df may be attributable to accumulated fibrin fibre orientation under the unidirectional flow component of CSPS. The LSCM and SEM images do not suggest significant fibrin fibre orientation in the CSPS results due, presumably, to the low levels of shear stress involved in the CSPS experiments and the low levels of shear elasticity associated with the incipient clot. However the results provide evidence of enhanced fibre bundling under flow, a feature which has been reported for mature clot microstructures [3, 17, 27].
5Conclusions
The results of this first study of blood coagulation by CSPS suggest that it is a valuable haemorheological tool in the study of modified clot microstructure. They also lend further support to the hypothesis that df predominantly reflects the fibrin network arrangement, and its role as the principal microstructural component of the incipient clot [14]. The results illustrate the relationship between this microstructural parameter and a mechanical property (shear elasticity) underlying clot haemostatic functionality. Moreover, the elevation of df above the values previously attained in fibrin clots by increasing thrombin concentration is evidence of the ability of unidirectional flow to enhance activation and clot assembly, leading to the incorporation of more polymerized mass within the incipient clot. This feature, along with the corresponding elevation of incipient clot elasticity, may have clinical significance in terms of modified clot structure in disease states [1]. Instances of incipient clot formation featuring substantially enhanced mass and elasticity clearly merit further study. Since even the relatively low shear rates studied here have significant effects on clot microstructure and mechanical properties, the much greater shear rates encountered in stenotic vessels associated with thrombosis could have even more striking effects.
Acknowledgments
This work was supported by EPSRC(UK) grants EP/C513037/ 1 and EP/I019405/1 and a NISCHR BRU award.
References
1 | Blomback B, Bark N(2004) Fibrinopeptides and fibrin gel structureBiophys Chemist112: 147151 |
2 | Booij HC(1966) Influence of superimposed steady shear flow on the dynamic properties on non-Newtonian fluids I: Measurements on non-Newtonian solutionsRheol Acta5: 215221 |
3 | Campbell RA, Aleman M, Gray LD, Falvo MR, Wolberg AS(2010) Flow profoundly influences fibrin network structure: Implications for fibrin formation and clot stability in haemostasisThromb Haemost104: 12811284 |
4 | Carvalho W, Djabourov M(1997) Physical gelation under shear for gelatin gelsRheol Acta36: 591609 |
5 | Chambon F, Winter HH(1987) Linear viscoelasticity at the gel point of a crosslinking PDMS with imbalanced stoichiometryJ Rheol31: 683697 |
6 | Cheng CP, Herfkens RJ, Taylor CA(2003) Inferior vena caval hemodynamics quantified in vivo at rest and during cycling exercise using magnetic resonance imagingAm J Physiol Heart Circ Physiol284: H1161H1167 |
7 | Colace TV, Muthard RW, Diamond SL(2012) Thrombus growth and embolism on tissue factor-bearing collagen surfaces under flow role of thrombin with and without fibrinArterioscler Thromb Vasc Biol32: 14661476 |
8 | Collet JP, Allali Y, Lesty C, Tanguy ML, Silvain J(2006) Altered fibrin architecture is associated with hypofibrinolysis and premature coronary atherothrombosisArterioscler Thromb Vasc Biol26: 25672573 |
9 | Collet JP, Lesty C, Montalescot G, Weisel JW(2003) Dynamic changes of fibrin architecture during fibrin formation and intrinsic fibrinolysis of fibrin-rich clotsJ Biol Chem278: 2133121335 |
10 | Collet JP, Park D, Lesty C, Soria J, Soria C, Montalescot G(2000) Influence of fibrin network conformation and fibrin fiber diameter on fibrinolysis speed: Dynamic and structural approaches by confocal microscopyArterioscler Thromb Vasc Biol20: 13541361 |
11 | Curtis DJ, Brown MR, Hawkins K, Evans PA, Lawrence MJ, Rees P, Williams PR(2011) Rheometrical and molecular dynamics simulation studies of incipient clot formation in fibrin-thrombin gels: An activation limited aggregation approachJ Non-Newton Fluid Mechanics166: 932938 |
12 | Curtis DJ, Williams PR, Badiei N, Campbell AI, Hawkins K, Evans PA, Brown MR(2013) A study of microstructural templating in fibrin–thrombin gel networks by spectral and viscoelastic analysisSoft Matter9: 48834889 |
13 | Evans PA, Hawkins K, Lawrence M, Williams RL, Barrow MS, Thirumalai N, Williams PR(2008) Rheometry and associated techniques for blood coagulation studiesMed Eng Phys30: 671679 |
14 | Evans PA, Hawkins K, Morris RHK, Thirumalai N, Munro R, Wakeman L(2010) Gel point and fractal microstructure of incipient blood clots are significant new markers of hemostasis for healthy and anticoagulated bloodBlood116: 33413346 |
15 | Evans PA, Hawkins K, Williams PR, Williams RL(2008) A study of gelatin gelation by Fourier transform mechanical spectroscopyJ Non-Newtonian Fluid Mechanics148: 122126 |
16 | Ferri F, Greco M, Arcovito G, De M(2002) Spirito and M. Rocco, Structure of fibrin gels studied by elastic light scattering techniques: Dependence of fractal dimension, gel crossover length, fiber diameter, and fiber density on monomer concentrationPhys, Rev E66: 011913 |
17 | Gersh KC, Edmondson KE, Weisel JW(2010) Flow rate and fibrin fiber alignmentJ Thromb Haemost8: 28262828 |
18 | Glover CJ, McIntire LV, Leverett LB, Hellums JD, Brown CH, Natelson EA(1974) Effect of shear stress on clot structure formationASAIO Journal20: 463468 |
19 | Hawkins K, Evans PA, Lawrence M, Curtis D, Davies TM, Williams PR(2010) The development of rheometry for strain-sensitive gelling systems and its application in a study of fibrin-thrombin gel formationRheol Acta49: 891900 |
20 | Jung F, Braune S, Lendlein A(2013) Haemocompatibility testing of biomaterials using human plateletsClin Hemorheol Microcirc53: 97115 |
21 | Kroll MH, Hellums JD, Mclntire LV, Schafer A, Moake JL(1996) Platelets and shear stressBlood88: 15251541 |
22 | Lawrence MJ, Kumar S, Hawkins K, Bodend S, Rutt H, Mills G, Sabra A, Morris RHK, Davidson SJ, Badiei N, Brown MR, Williams PR, Evans PA(2014) A new structural biomarker that quantifies and predicts changes in clot strength and quality in a model of progressive haemodilutionThrombosis Research134: 488494 |
23 | Lawrence MJ, Sabra A, Mills G, Pillai SG, Abdullah W, Hawkins K, Morris RHK, Davidson SJ, D’Silva LA, Curtis DJ, Brown MR, Wiesel JW, Williams PR, Evans PA(2014) A new biomarker quantifies differences in clot microstructure in patients with venous thromboembolismBritish Journal of Haematology10.1111/bjh.13173 |
24 | Mills JD, Ariens RA, Mansfield MW, Grant PJ(2002) Altered fibrin clot structure in the healthy relatives of patients with premature coronary artery diseaseCirculation106: 19381942 |
25 | Monroe DM, Hoffman M, Roberts HR(2002) Platelets and thrombin generationArterioscler Thromb Vasc Biol22: 13811389 |
26 | Mosesson M(2005) Fibrinogen and fibrin structure and functionsJ Thrombosis and Haemostasis3: 18941904 |
27 | Muenster S, Jawert LM, Fabry B, Weitz DA(2013) Structure and mechanics of fibrin clots formed under mechanical perturbationJ Thromb Haemost11: 557560 |
28 | Muthukumar M(1989) Screening effect on viscoelasticity near the gel pointMacromolecules22: 46564658 |
29 | Neeves KB, Illing DA, Diamond SL(2010) Thrombin flux and wall shear rate regulate fibrin fiber deposition state during polymerization under flowBiophys J98: 13441352 |
30 | Piechocka IK, Bacabac RG, Potters M, MacKintosh FC, Koenderink GH(2010) Structural hierarchy governs fibrin gel mechanicsBiophysical Journal98: 22812289 |
31 | Reinhart WH(2013) Platelets in vascular diseaseClin Hemorheol Microcirc53: 7179 |
32 | Ryan EA, Mockros LF, Weisel JW, Lorand L(1999) Structural origins of fibrin clot rheologyBiophys J77: 28132826 |
33 | Sander LM(1986) Fractal growth processesNature322: 789793 |
34 | Scanlan JC, Winter HH(1991) Composition dependence of the viscoelasticity of end-linked poly(dimthylsiloxane) at the Gel PointMacromolecules24: 4754 |
35 | Somma E, Valentino O, Titomanlio G, Ianniruberto G(2007) Parallel superposition in entangled polydisperse polymer melts: Experiments and theoryJ Rheol51: 987 |
36 | Undas A, Ariens RA(2011) Fibrin clot structure and function: A role in the pathophysiology of arterial and venous thromboembolic diseasesArterioscler Thromb Vasc Biol31: 8899 |
37 | Varju I, Sotonyi P, Machovich R, Szabo L, Tenekedjiev K, Silva MMCG(2011) Hindered dissolution of fibrin formed under mechanical stressJ Thromb Haemost9: 979986 |
38 | Weisel J(2004) The mechanical properties of fibrin for basic scientists and cliniciansBiophys Chemist112: 267276 |
39 | Weisel JW, Litvinov RI(2013) Mechanisms of fibrin polymerization and clinical implicationsBlood11: 17121719 |
40 | Weitz DA, Oliveria M(1984) Fractal structures formed by kinetic aggregation of aqueous gold colloidsPhysical Review Letters52: 1433 |
41 | Whittaker P, Przyklenk K(2009) Fibrin architecture in clots: A quantitative polarized light microscopy analysisBlood Cells Mol Dis42: 5156 |
42 | Young D, Bermes E(1999) Specimen collection and processing sources of biological variationTietz textbook of clinical chemistryBurtis CA, Ashwood ERPart 3: 4272WB SaundersPhiladelphia |
Figures and Tables
Fig.1
Illustration of CSPS. The technique is used to apply combined unidirectional and oscillatory shear stresses (σs and σo, respectively), the direction of the two resulting shear motions (i.e. the unidirectional and oscillatory components) being parallel (see Fig. 1(i)). The net accumulated unidirectional strain is zero under SAOS whereas it increases progressively in CSPS (Fig. 1(ii)). The SAOS strain decreases progressively in blood as the GP is approached. CSPS involves a time-varying shear rate under constant stress due to rheological changes associated with clotting. The unidirectional flow shear rate becomes vanishingly small in the vicinity of the GP.
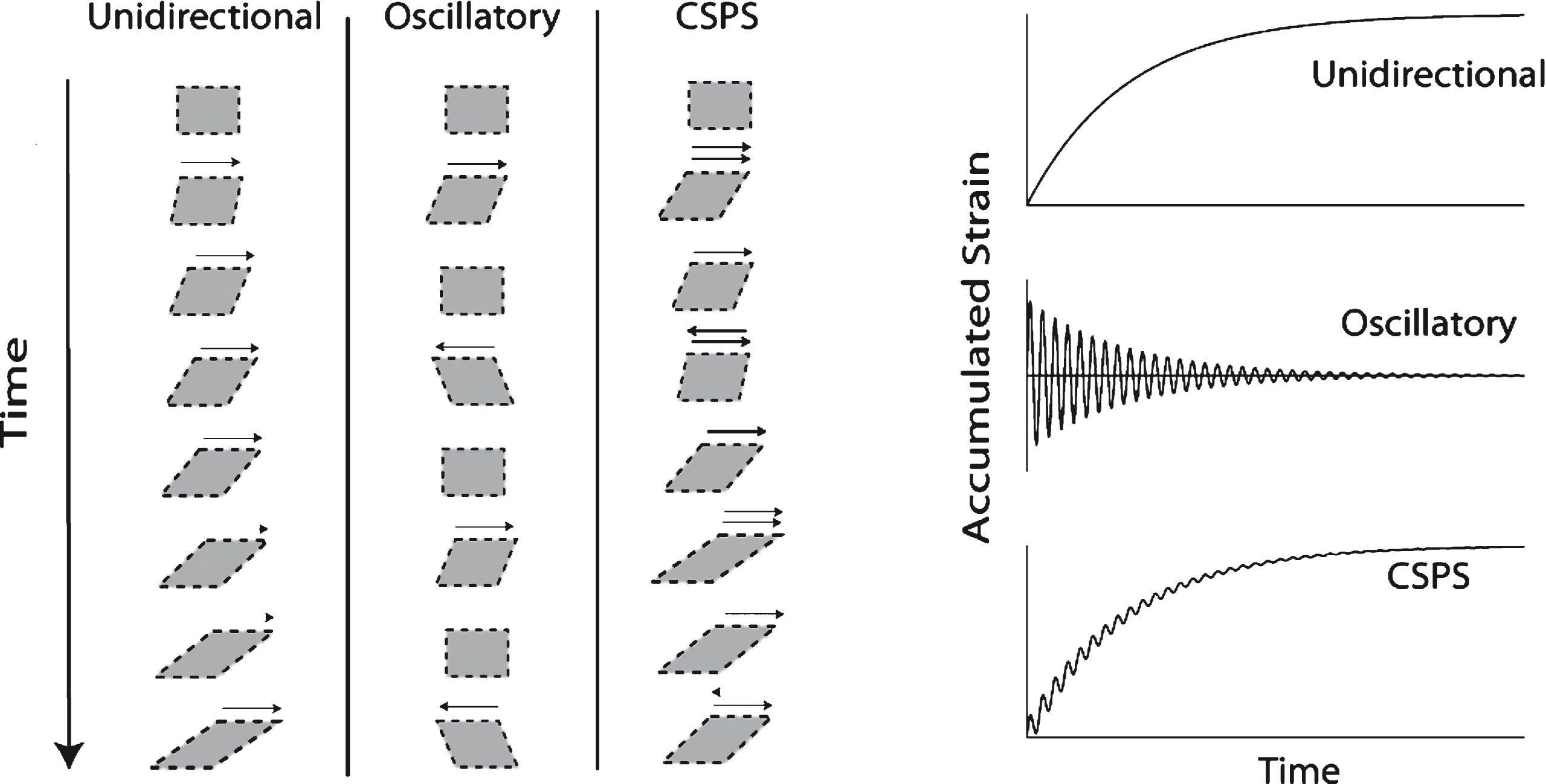
Fig.2
Variation of gel time (left) and fractal dimension (right) for increasing levels of unidirectional shear stress in blood (squares) and fibrin thrombin gels (circles).
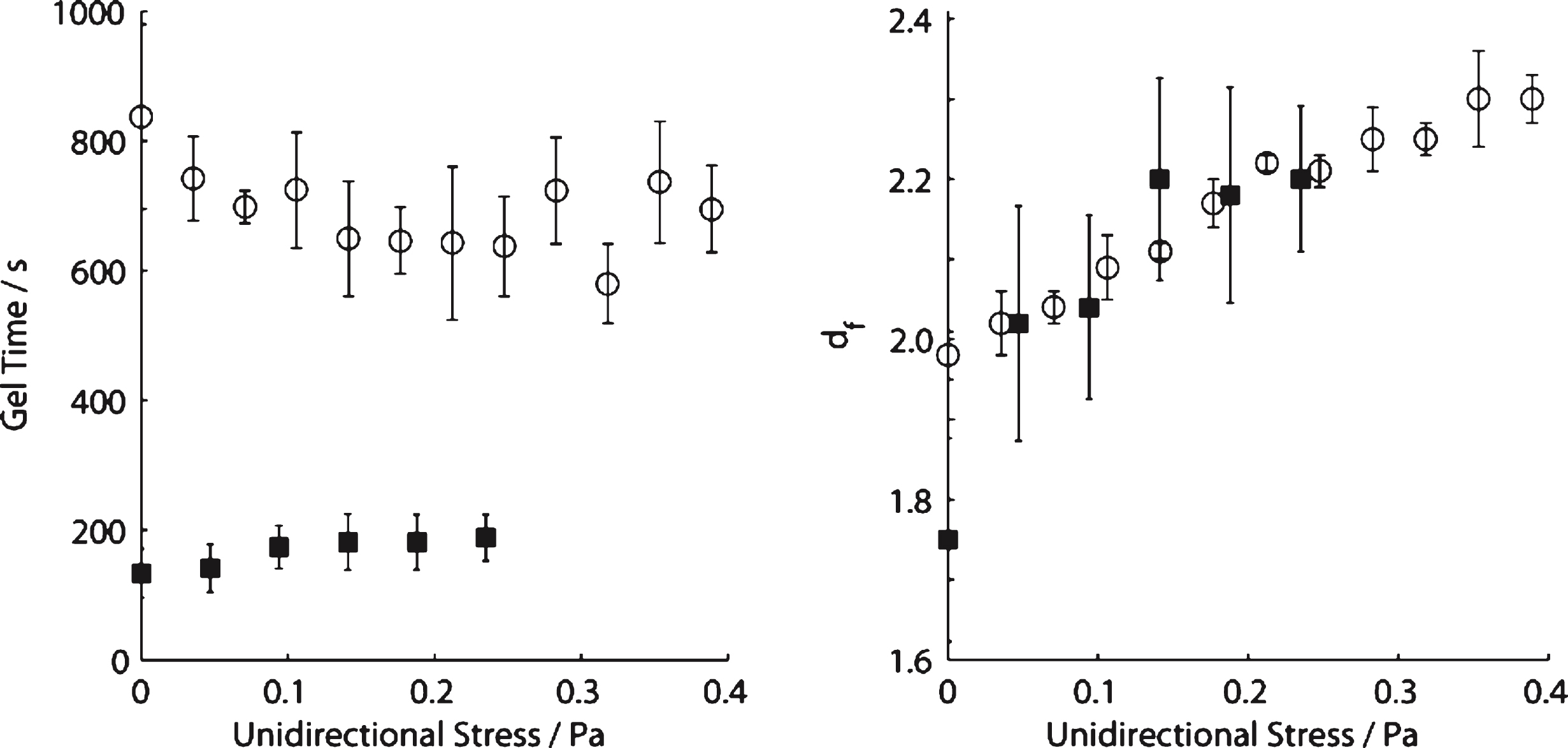
Fig.3
CSPS results for whole blood. The unidirectional shear stress is σs= 0.047 Pa. The frequency independent value of δ marks the GP and the establishment of an incipient clot characterized by df= 2.0, significantly higher than that recorded under SAOS (df= 1.75). The maximum value of the unidirectional shear rate
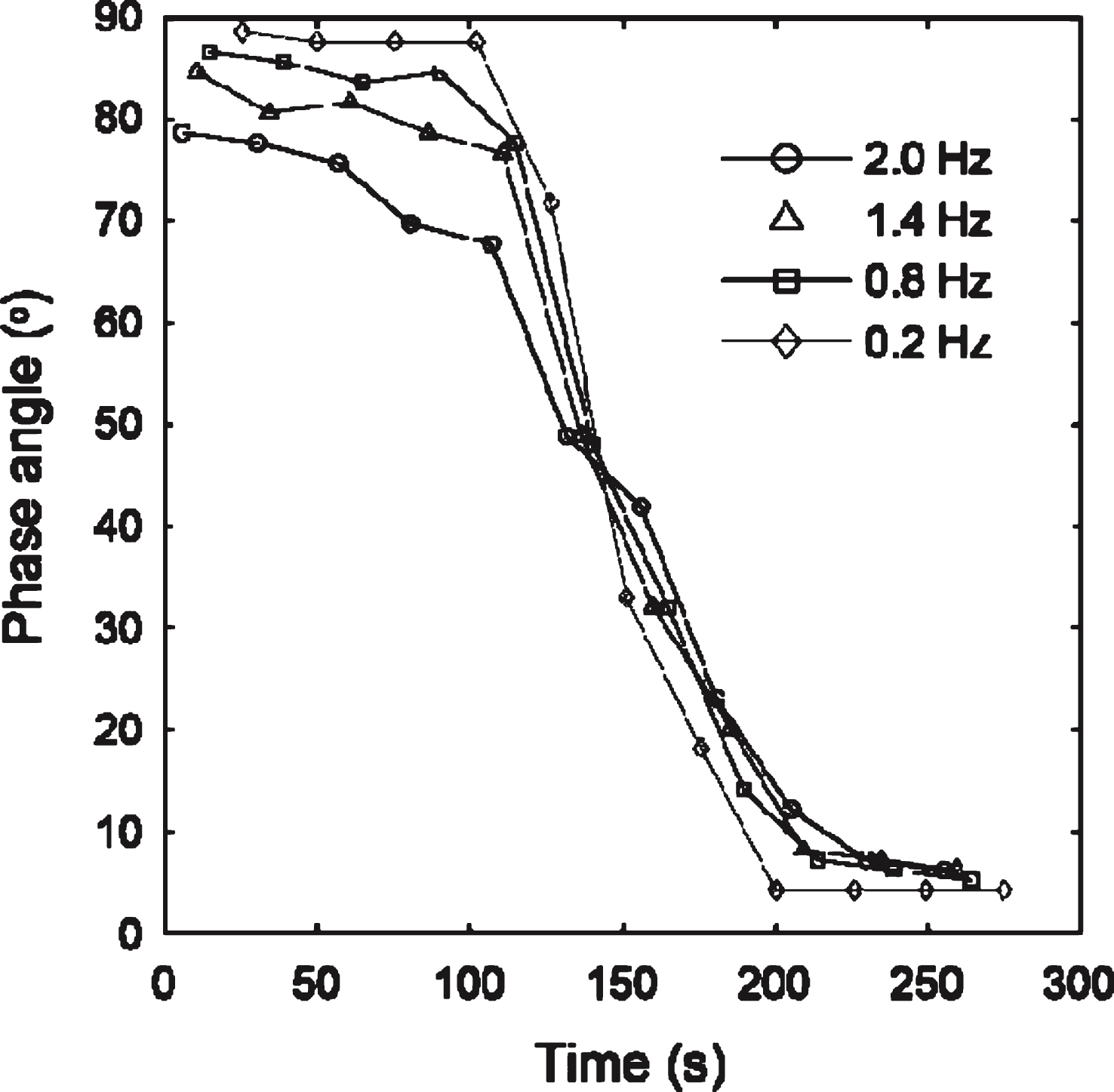
Fig.4
Values of elastic modulus, G′ (circles) and loss modulus G′′ (triangles) obtained as a function of angular frequency in SAOS tests on a fibrin-thrombin gel at the GP. Inset shows the corresponding frequency independent values oftan δ (= G′′/G′) for a fibrin gel formed under SAOS (open triangles) and CSPS with σ= 0.177 Pa (open circles). The results reveal a decrease in tan δ (corresponding to an increase in df) under CSPS relative to SAOS.
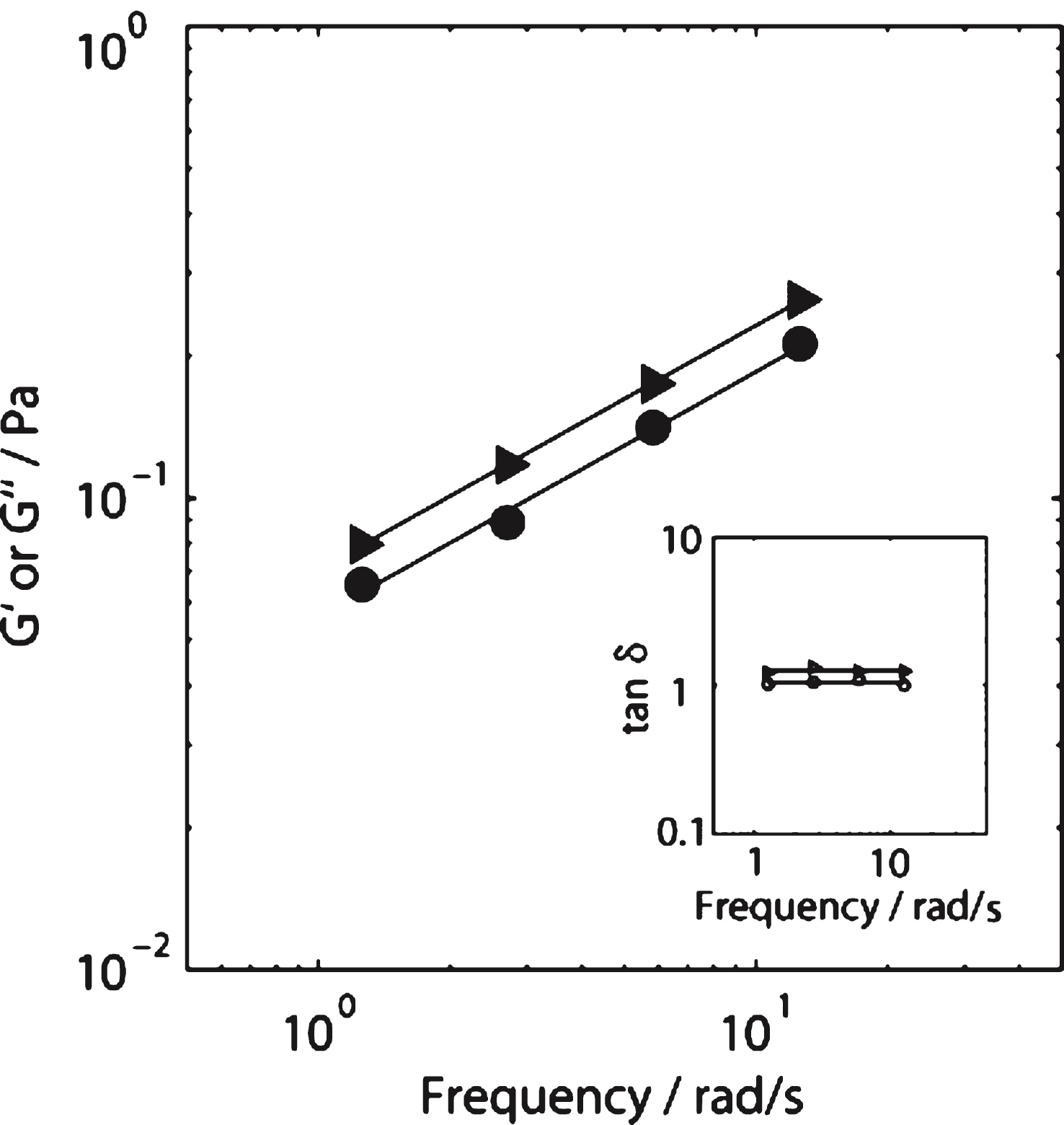
Fig.5
Structure-function relationship in terms of df and incipient clot elasticity for whole blood (CSPS) and fibrin gels (in SAOS and CSPS tests). The values of G′GP and
![Structure-function relationship in terms of df and incipient clot elasticity for whole blood (CSPS) and fibrin gels (in SAOS and CSPS tests). The values of G′GP and G∥GP′ are normalized by their respective values at df= 2.0. The SAOS results for fibrin gels result from a progressive increase in thrombin concentration at a fixed value of fibrinogen concentration [φ= 0.01 to 0.19 NIH/ml, c = 10 mg/ml].](https://ip.ios.semcs.net:443/media/ch/2015/60-4/ch-60-4-ch1924/ch-60-4-ch1924-g005.jpg)
Fig.6
LSCM images of a fibrin gel formed under SAOS and those formed under CSPS at three different levels of shear stress. Fibrin gel network formed under (A) SAOS (σs= 0 Pa), (B) CSPS (σs= 0.1 Pa) and (C) CSPS (σs= 0.35 Pa) with the corresponding values of df being 1.99 (± 0.01), 2.03 (± 0.01) and 2.3 (± 0.05), respectively. The images were acquired immediately following the attainment of the GP in the rheometer. The scale bar width is 20 μm.
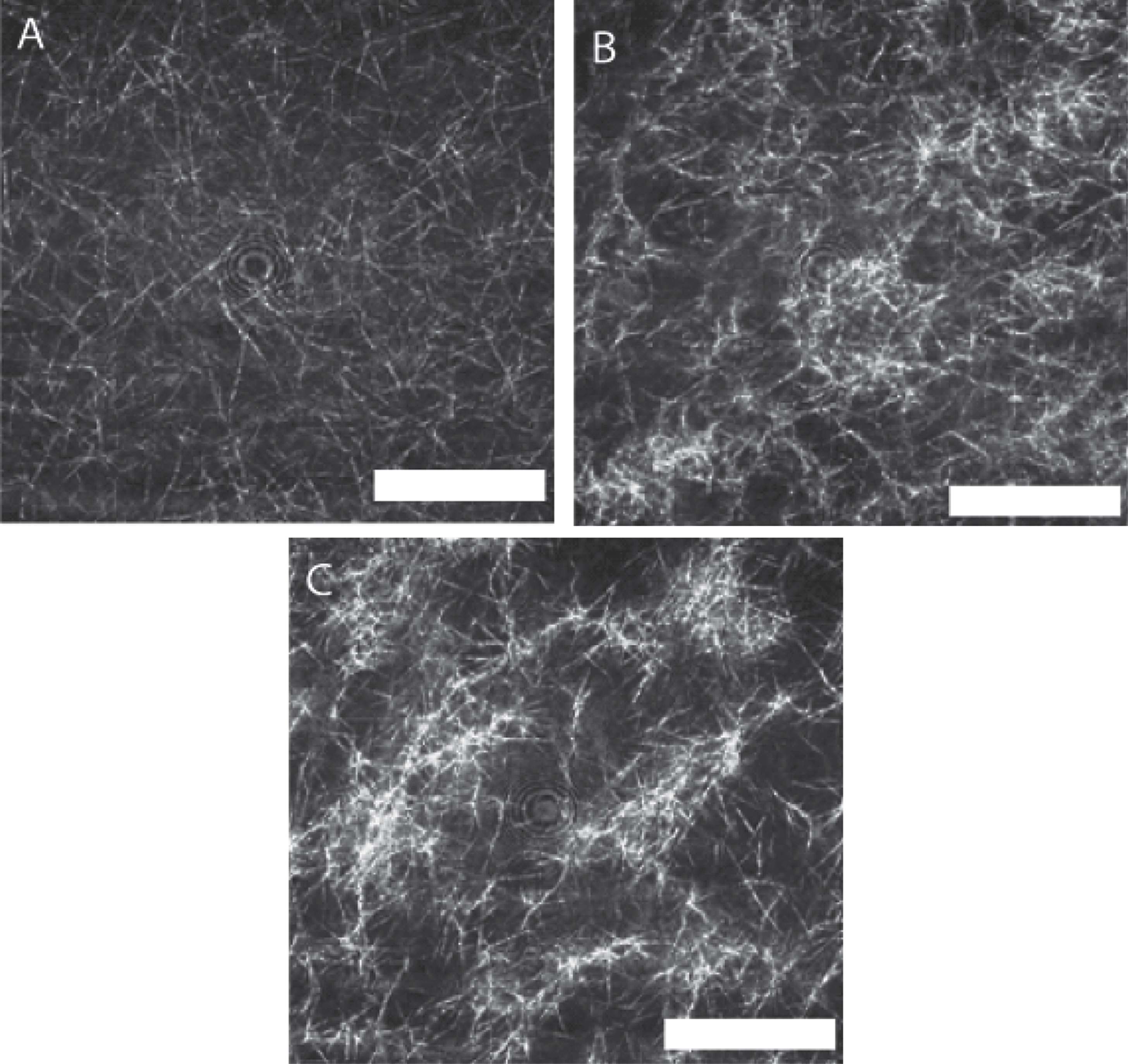
Fig.7
SEM images of a fibrin gel formed under SAOS and those formed under CSPS at different levels of shear stress. Fibrin gel network formed under (A) SAOS (σs= 0 Pa), (B) CSPS (σs= 0.1 Pa) and (C) CSPS (σs= 0.35 Pa) with the corresponding values of df being 1.99 (± 0.01), 2.03 (± 0.01) and 2.3 (± 0.05), respectively. The scale bar width is 5 μm.
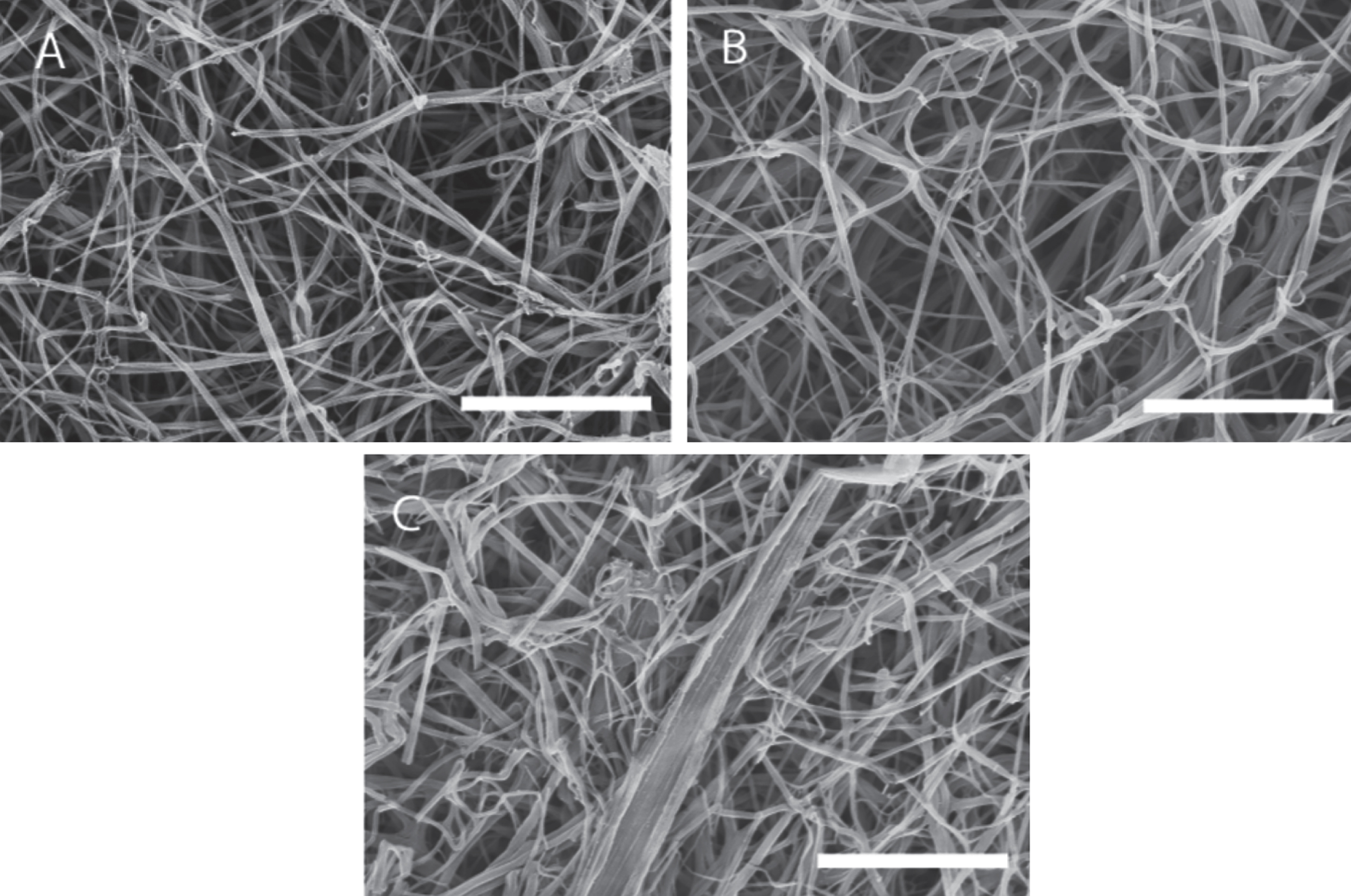
Fig.8
Measurements of fibrin fibre diameter from analysis of SEM images of clots formed under SAOS (lower, σs= 0 Pa) and different levels of applied unidirectional shear stress in CSPS.
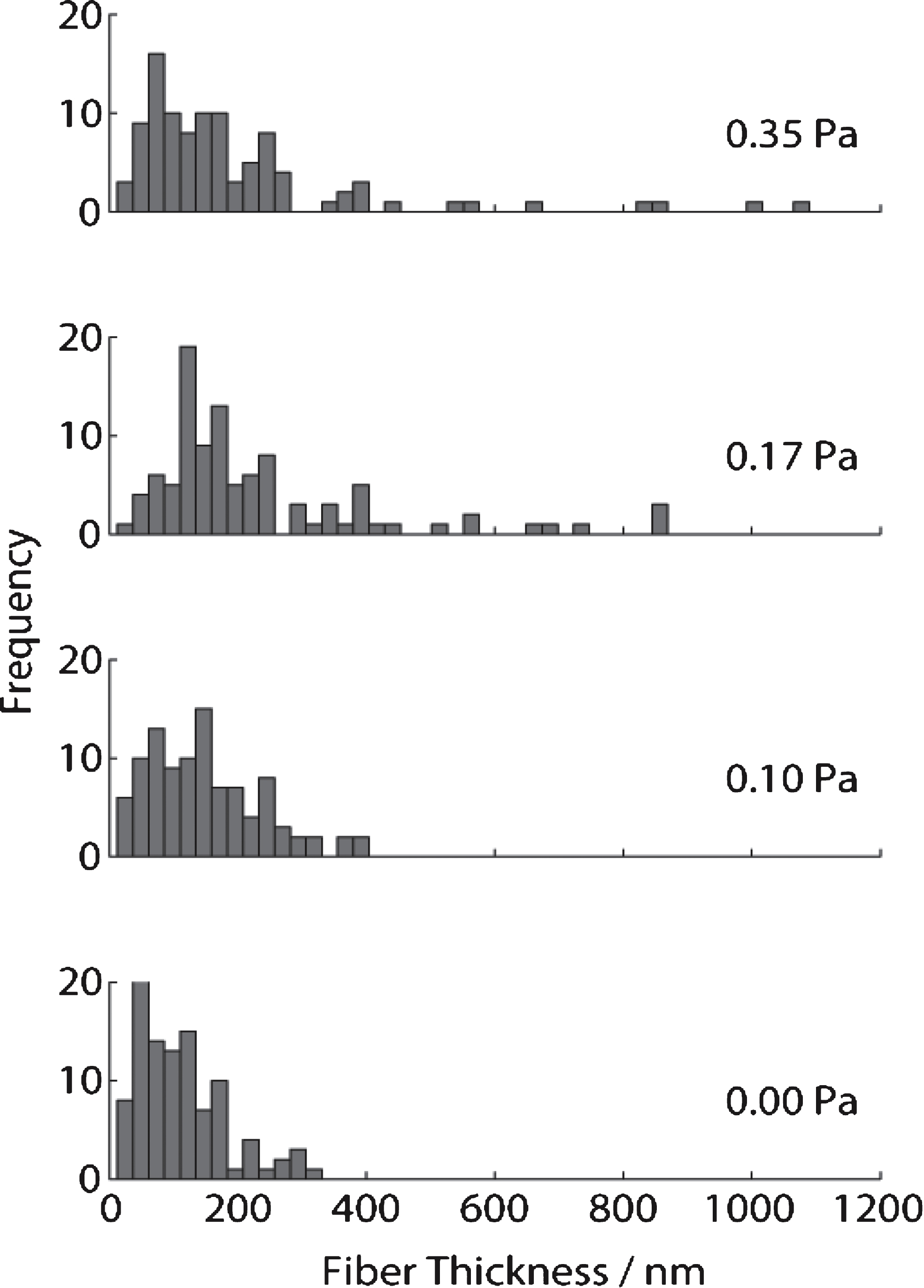