Cholesterol: Revisiting its fluorescent journey on 200th anniversary of Chevruel’s “cholesterine”
Abstract
The legacy of Micheal Chevruel’s discovery of “cholesterine” as a non-saponifiable lipid from gall stones has ignited the imagination and research of countless minds for over two centuries now. In this review, we have provided a brief chronicle of the early history of cholesterol research which paved the way to present day understanding of membrane biology. We have discussed the properties and functionality of various fluorescent analogs of cholesterol in view of the ultra-high sensitivity, rapid response and spatial resolution obtained using fluorescence spectroscopic, microscopic and flow cytometric techniques. The repertoire of fluorescent analogs discussed for cholesterol research include the naturally occurring analogs (dehydroergosterol and cholestatrienol); polarity sensitive probes (NBD- and dansyl-cholesterol); bright and photostable probe (BODIPY-cholesterol); clickable alkyne cholesterol and cholesterol binding macromolecules (fluorescently labeled non-toxic subunits of perfringolysin O and filipin) in monitoring cholesterol content in live and fixed cells. We have elaborated on the applications of the fluorescent analogs of cholesterol in clinical research, taking atherosclerosis, Niemann–Pick C and Alzheimer’s disease as representative examples. The applicability of fluorescent probes of cholesterol has become more relevant with the advent of various super-resolution microscopic techniques today and holds the promise of shedding light into the molecular orchestra of lipid-protein interaction with nanometer-scale resolution.
1.Cholesterol, a “Nobel” molecule: A brief journey through its fascinating history
Cholesterol is a representative non-polar lipid in higher eukaryotic cellular membranes and is crucial for membrane organization, dynamics, function, and vesicular sorting [89,112,150]. Cholesterol is a predominantly hydrophobic molecule comprising of a near planar tetracyclic fused steroid ring and a flexible isooctyl hydrocarbon tail [see Fig. 1]. The isolation of crystals of cholesterol from gall-stones was first performed by Lyon-born doctor François Poulletier de la Salle in 1758 by dissolving powdered gall-stones in warm alcohol [84]. Poulletier identified cholesterol as a form of wax, known through his personal communications with Pierre-Joseph Macquer, who documented the finding in Dictionnaire de Chymie in 1788 [84]. Poulletier also communicated his results to Fourcroy, who gave a more detailed account in 1789 after the Poulletier’s demise [11]. Between 1775–1789, the isolation of “shiny” crystalline material from gall stones was documented in multiple academic institutions in Europe. The first documentation was by Conradi in 1775 followed by Delius (1782) and Dietrich (1788) [84]. Delius and Dietrich, working with Gren, gave an early analysis of gallstones as a 85% waxy and 15% resinous material [84]. The first nomenclature of cholesterol came in 1815, when Michel Chevreul working in Paris recognized that fatty appearing substance from human gall stones could not be saponified, as mentioned by Poulletier, and he named it ”Cholesterine” from
Fig. 1.
Molecular anatomy of cholesterol and its influence in health and disease. (a) Chemical structure of cholesterol depicting its three structurally distinct regions, each outlined in a shaded box: a polar 3β-hydroxyl group (enclosed in the circle at the bottom), a rigid near-planar tetracyclic sterol ring (enclosed in the bigger box in the middle), and a flexible acyl chain (enclosed in the smaller box at the top). Historically, Michel Chevruel isolated cholesterol from gall stones and coined the term “cholesterine” to describe it for the first time in 1815. Currently, pure cholesterol (greater than 99% pure by GC chromatography) is commercially available from companies such as Sigma for research purposes from saponifation of lanolin extracted from sheeps’s wool. (b) Number of publications related to cholesterol per year from 1901 onwards. Data was obtained by searching Pubmed (http://www.ncbi.nlm.nih.gov/pubmed/) with the term “cholesterol”. The figure shows a steady increase in the number of publications in cholesterol from 1960’s onward. This coincides with the era when the chemical structure was determined and the biosynthetic pathway recently deciphered (see Fig. 2 and Section 1 in text for details). There has been an almost exponential increase in the number of papers published on cholesterol, indicating its enormous importance in a large number of field associated with health and diseases.
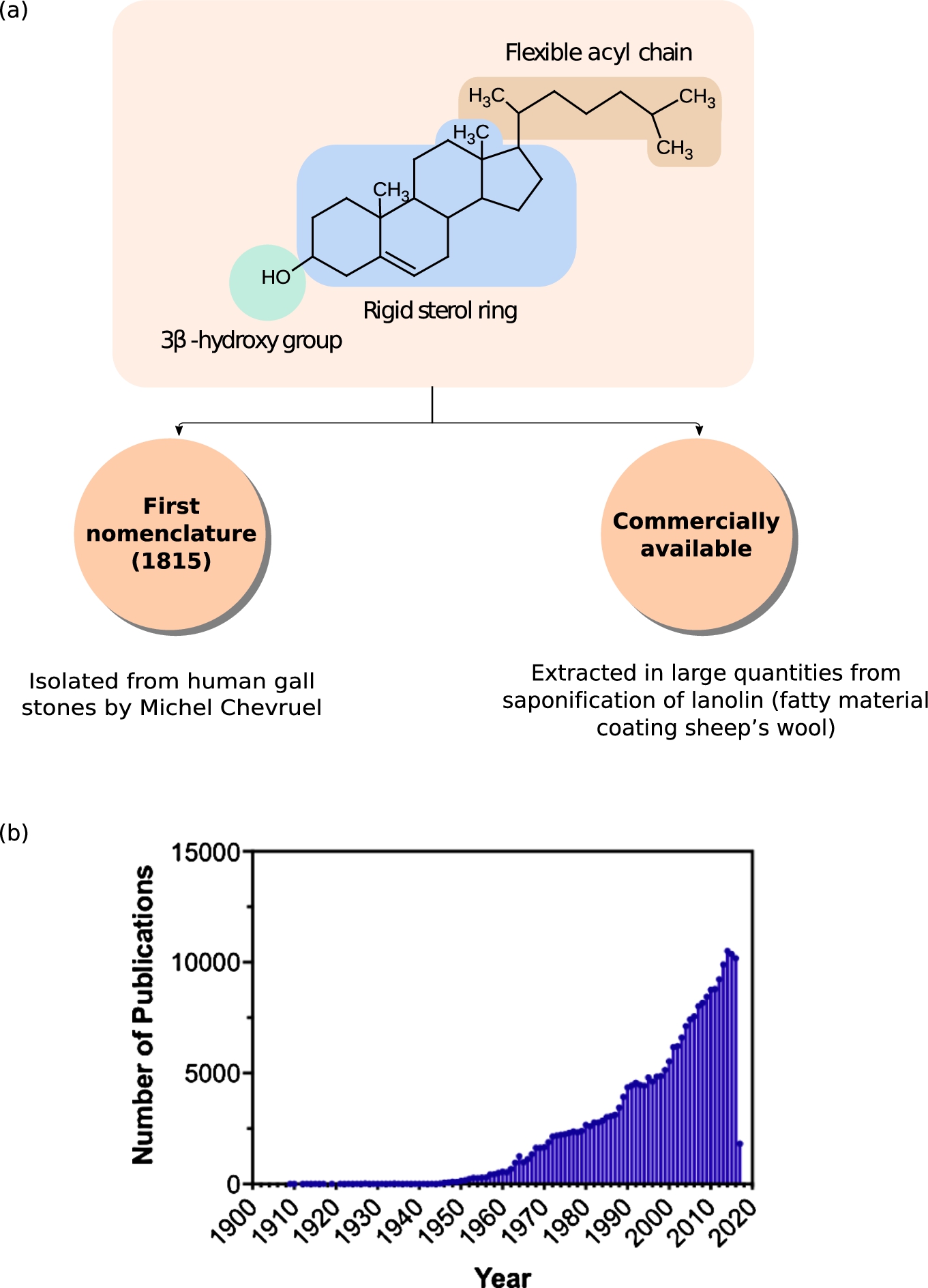
Fig. 2.
Cholesterol research and Nobel Prizes, an affair to last. The time line shows the chronological order of Nobel prizes conferred to researchers who have been involved in cholesterol research at some point in their life. The material for the timeline has been obtained from references [17,82,113] and from the Nobel lectures in www.nobelprize.org, see Section 1 in text for details.
![Cholesterol research and Nobel Prizes, an affair to last. The time line shows the chronological order of Nobel prizes conferred to researchers who have been involved in cholesterol research at some point in their life. The material for the timeline has been obtained from references [17,82,113] and from the Nobel lectures in www.nobelprize.org, see Section 1 in text for details.](https://ip.ios.semcs.net:443/media/bsi/2017/6-1-2/bsi-6-1-2-bsi166/bsi-6-bsi166-g002.jpg)
O.P.H. Diels ignited a volley of interest into the determination of cholesterol molecular structure around 1902, by identifying dicarboxylic acid as the end-product from reaction of cholesterol with NaOBr; and cholestenone derived from catalytic dehydration of cholesterol. He was later awarded the Nobel prize in 1950 for his work on diene synthesis by which unsaturated cyclic compounds could be produced. The leads from his research were robustly followed up by H.O. Wieland and A.O.R. Windaus in Germany, who received the Nobel Prize in Chemistry in 1928 for providing the first molecular structure of cholesterol. In 1933 however, Bernal, Rosenheim, Wieland and Windau published an alternate, correct structure, replacing the 1928 structure.
In mid 1920s, L. Ruzicka and his colleagues working in Switzerland on terpenes (extracted from perfumes) realized that “isoprene” is the common building block of cholesterol and the terpenes. This allowed L. Ruzicka to manipulate cholesterol structure toward the synthesis of male sex hormones, androsterone and testosterone. He was awarded the Nobel prize in 1939 for his work on polymethylenes and higher terpenes. R. Robinson working in Oxford University, embarked on the complete synthesis of cholesterol from 1939 onwards with his Australian student J.W. Cornforth and his wife Rita Cornforth. R. Robinson was awarded the Nobel Prize for his investigations on plant products of biological importance, especially the alkaloids which include chemicals like morphine, papaverine and narcotine in 1947. J.W. Cornforth worked on cholesterol synthesis project through his Ph.D. and finally established the full synthetic pathway in 1951. At about the same time, R.B. Woodward, working in Harvard University with his postdoctoral students, many of whom were funded from drug industries such as Merck and Monsanto, pioneered the stereo-chemical synthesis of cholesterol [82]. He was awarded the Nobel Prize in Chemistry in 1965 for his contribution to the organic synthesis of a large number of compounds including quinine, cholesterol, cortisone, strychnine, lysergic acid, reserpine, chlorophyll, cephalosporin, and colchicine.
After the establishment of cholesterol chemical synthesis, came the era of investigations into the bio-synthesis of the molecule. K. Bloch and F. Lynen were awarded the Nobel Prize in Physiology or Medicine in 1964 for their landmark studies of the cholesterol biosynthetic pathway, a complex sequence involving at least 30 steps. This was followed by D.H.R. Barton and O. Hassel establishing the “all chair” conformation of cholesterol and were awarded the Nobel Prize in Chemistry in 1969. J.W. Comforth, who had earlier synthesized cholesterol in R. Robinson’s laboratory, later collaborated with G. Popjak to establish the orientation of all of the hydrogen atoms in the cholesterol molecule, and received the Nobel Prize in Chemistry in 1975. M.S. Brown and J.L. Golstein working in University of Texas on familial hypercholesterolemia patients discovered the receptor for low-density lipoprotein, or LDL, which carries cholesterol in blood. They were awarded the Nobel Prize in 1985 for their discoveries concerning the regulation of cholesterol metabolism. This “midas touch” of cholesterol continues with the very recent Nobel prize for studies on G protein-coupled receptors (GPCRs). The X-ray crystal structure of β-2-adrenergic receptor (the first purified and characterized GPCR that stimulates via adenylate cyclase) that provided a wealth of information into the molecular architecture of GPCRs in general, was co-crystallized “cholesterol” [28].
Since the 1970’s there has been interest in developing and applying modern spectroscopic methods as a means to study the structure and function of biological membranes [178]. Fluorescence techniques in particular, provide a variety of unique advantages such as (i) high sensitivity of detection resulting in low concentrations of reporter molecules, minimizing the impact of the probe on the behavior of the system, (ii) the significant response of a number of fluorescence parameters (such as intensity, anisotropy, lifetime) to the physical and chemical changes of local environment, (iii) overlap of the intrinsic time scale of the fluorescence (∼ nanoseconds) with the time scale of many chemical and biochemical processes, (iv) the possibility of multi-parameter analysis arising from ratio-metric dual-band fluorophores [78]. The ultra-high sensitivity, the high speed of response, and the high spatial resolution expands the usage of the fluorescent probes as tracers for localization of biological structures by fluorescence microscopy, for quantitation of analytes by fluorescence immunoassay, for flow cytometric analysis of cells, for measurement of physiological state of cells and among other applications [26,51,158]. The founding of Molecular Probes in 1975 by Richard Paul and Rosaria Haugland made lipid probes accessible to a large cohort membrane researchers across the globe and propelled the advancement of cholesterol research with the ready availability of fluorescently labelled cholesterol.
Fig. 3.
Dehydroergosterol (DHE) and cholestatrienol (CTL), naturally occurring fluorescent sterols. A brief timeline tracing the chronology of the chemical synthesis of (a) DHE and (b) CTL and their application in membrane research is shown. Chemical structures and the commercial availability of both the cholesterol analogs are also provided. See Section 2.1 for details on the timeline.
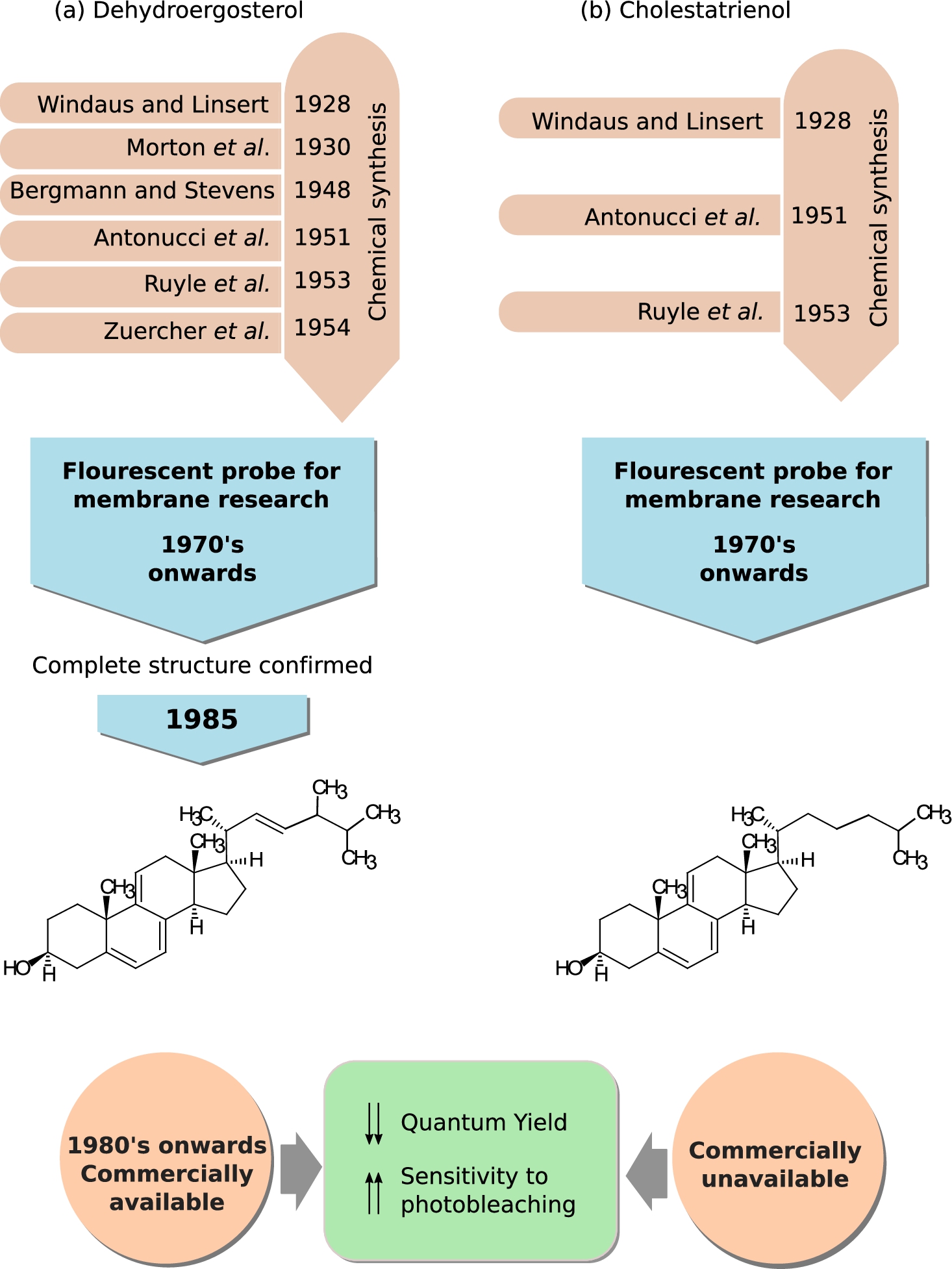
2.Fluorescent probes used in cholesterol research
2.1.Naturally occuring fluorescent sterols
The earliest fluorescent probes in cholesterol research were the two naturally occuring forms of cholesterol, dehydroergosterol (DHE) and cholestatrienol (CTL) [99,102,154,171]. The fluorescence properties of these sterols (in the near UV-region of the spectrum) stem from the three conjugated double bonds in their steroid ring [99]. DHE is closer in structure to ergosterol, a sterol found in cell membranes of fungi and protozoa, compared to CTL which has more similarity to cholesterol. Both these sterols have been used as cholesterol mimetics in membrane studies from as early as 1975 [5,136] to present [63,108]. Figure 3 shows a brief time line embodying the early synthesis of DHE and CTL, use in membrane biology, and their chemical structure.
DHE was first chemically synthesized in 1929 [40] by Adolf Winduas (first Nobel prize winner in cholesterol research) and his group, although its discovery from natural sources such as Red Sea sponge Biemna fortis [36], yeast species like Candida tropicalis [149] and Saccharomyces cerevisiae [6] was as late as 1980’s. Despite its long pedigree, the complete chemical structure of DHE was not confirmed until 1985 [40], although it was being used in model membrane studies from 1975 onwards [136]. Earliest use of DHE as a fluorescent probe relied on its synthesis from ergosterol [10,40,136]. The first commercially available form of DHE was produced by Frann Scientific Inc. (Columbia, MO) [29] before it became available from other companies like Steraloids, Wilmington, NH; Aldrich Chemical Co., Milwaukee, WI; Fluka Chemie, Steinheim, Switzerland; Sigma Chemical, St Louis, MO [103].
The credit for the first chemical synthesis of CTL also goes to Adolf Winduas [168]. Figure 3(b) gives a brief account of the history of early synthesis of CTL, its use in membrane research and its chemical structure. The yield of CTL was greatly improved by modification of the original synthesis protocol in 1950s [4,137]. It’s use as a fluorescent probe in membrane biology started from 1970s onwards [136,141,152]. However, the use of CTL as a fluorescent probe is restricted by the lack of commercial availability. Currently, methods have been developed to synthesize it from DHE [136] but CTL is not widely available. Use of DHE [18,30,33,41,109,120,127,133,177] and CTL [30,109,127,141,177] in membrane research has been extensively reviewed in the last few years [99,102,154,171]. Their use in clinical research is discussed later in the review (see Section 2.3).
2.2.Acyl chain modified fluorescent cholesterol
2.2.1.NBD-cholesterol and dansyl-cholesterol
Solvatochromic fluorophores such as 7-Nitrobenz-2-oxa-1,3-diazol-4-yl (NBD) and 1-dimethylaminonaphthalene-5-sulphonic acid (Dansyl) exhibit distinct spectral shifts and alterations in fluorescence lifetime in response to changes in the polarity of the local environment. Ghosh and Whitehouse were the first to report the synthesis of NBD chloride in 1968 [47] and from 1970’s onwards it was a popular choice among other fluorescent probes for labelling lipids [23]. The NBD-group has been linked to two main positions on the cholesterol backbone: (i) in one case, NBD group is esterified to the hydroxyl group of cholesterol [1,135,145] and (ii) in the other, NBD group is covalently attached to the branched alkyl chain of cholesterol [24,25,67]. The hydroxyl head group of cholesterol acts as both hydrogen bond donor and acceptor in interaction with cholesterol molecules, other lipid (especially, sphingolipids) and interfacial water molecules [2,122]. The fluorescent probes where the NBD-group is esterified to the hydroxyl group of cholesterol are not good representatives of cholesterol in membranes both in terms of structural orientation and functional properties, because of the loss of the hydrogen bonding property.
There are two main classes of acyl modified fluorescent NBD-cholesterol, (i) 22-NBD-cholesterol and (ii) 25-NBD-cholesterol. 25-NBD cholesterol has a more intact alkyl chain compared to 22-NBD cholesterol, the seven-membered alkyl chain is truncated at the 17β-position in the latter. A concern with truncated chain length stems from the importance of a critical length of alkyl chain in cholesterol orientation obtained from detailed biophysical studies [105,115,139]. Both acyl forms of NBD-cholesterol probes have been extensively used in model and cell membrane studies for probing membrane physical properties and lipid-protein interaction. Their spectroscopic properties and applications in membrane research has been extensively reviewed earlier [26,52,70,102,171]. Figure 4(a) and (c) show the chemical structures of 22- and 25-NBD cholesterol. Some of the clinical applications of NBD-cholesterol are discussed later in the review (see Section 2.3).
Fig. 4.
Polarity sensitive fluorescent probes of cholesterol. NBD and Dansyl groups are solvatochromic fluorophores, whose fluorescence read-outs (such as emission maxima, lifetime, anisotropy) are sensitive to change in the polarity of the local environment. A brief timeline tracing the chemical synthesis of the probes NBD-Cl and Dansyl-Cl, followed by their conjugation to form (a) 25-NBD-cholesterol, (b) Dansyl-cholesterol and (c) 22-NBD-cholesterol and use in membrane research is provided. In addition, the chemical structures of these three analogs and information on their commercial availability is also shown.
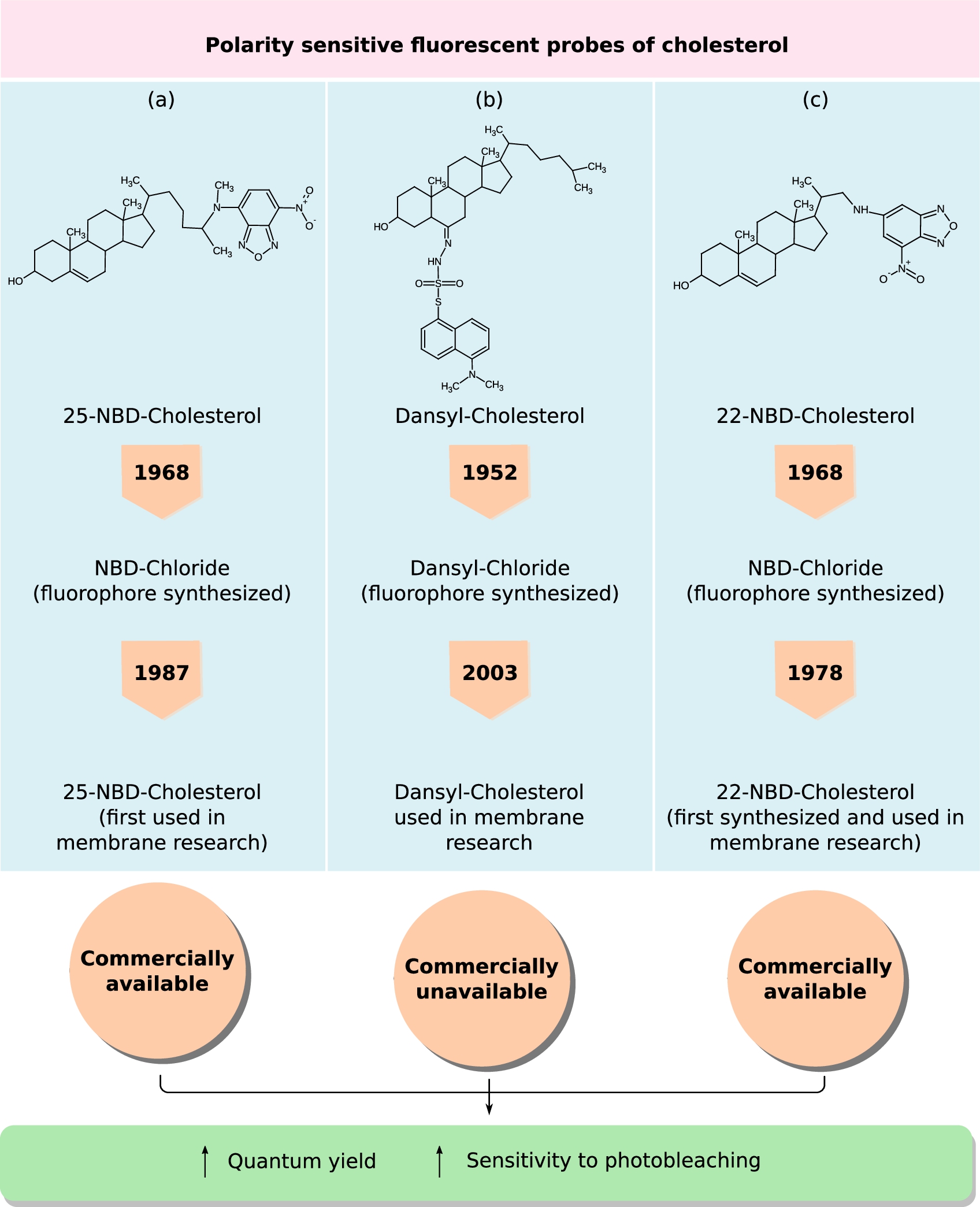
Dansyl-Chloride was synthesized earlier than NBD-Chloride by one of the greatest fluorescent spectroscopist of the century, Prof. Gregario Weber, in 1952 [163]. This fluorophore was originally synthesized for protein labeling and it’s usage evolved over time and in 2003 (50 years after its initial synthesis) it was covalently linked to the sixth carbon atom of the steroid backbone of cholesterol (D-chol) by Gimpl’s group [167]. A great advantage of D-Chol, like NBD-Cholesterol, is the very low quantum yield (
Figure 4(a)–(c) traces a brief chronicle embodying the discovery of the polarity-sensitive probes, synthesis of the cholesterol-derivatives and their availability to users.
2.2.2.BODIPY-cholesterol
Boron-containing diazaindacene fluorophores constitute a class of very bright and photostable probes with very low environmental sensitivity (relative to NBD and Dansyl probes) used in membrane research. The first fluorophore of this class was synthesized by Treibs and Kreuzer in 1968 [159]. Other derivatives of the fluorophore were synthesized later by Vos de Wael et al. in 1977 [35] and Worries et al. in 1985 [170]. However, their research did not provide methods where the fluorophores could be chemically reacted with ligands. This vacuum was filled by the synthesis of various reactive form of the fluorophore, dipyrrometheneboron difluoride (under the trade name BODIPY), by Haugland and Kang from Molecular Probes in 1988 [56]. The probe was conjugated to many fatty acids and proteins [68] and it was in 2006 that a BODIPY-cholesterol analog joined the league [83]. The BODIPY fluorophore conjugated at carbon 24 of the cholesterol sterol ring (see Fig. 5) is the most functional reporter among a series of other conjugated forms synthesized by Bittman and co-workers [96] owing to the importance of longer alkyl chain length [105,115,139]. This was followed by the commercial availability of BODIPY-conjugated cholesterol under the trade name TopFluor-cholesterol (Avanti Polar Lipids) from 2009 onwards (personal communication with Dr. Stephen Burgess, Avanti Polar Lipids). BODIPY-cholesterol offers superior photo-physical properties such as a high molar extinction coefficient (
Fig. 5.
BODIPY-Cholesterol, a bright and photostable fluorescent probe of cholesterol. BODIPY falls under the class of boron-containing diazaindacene fluorophores, which are bright and photostable probes with very low environmental sensitivity (relative to NBD and Dansyl probes) used in membrane research. The figure shows the chemical structure of the most promising and functional variant of BODIPY-cholesterol (see Section 2.2.2 for details). A chronological timeline of the chemical synthesis of the fluorescent probe, BODIPY and its attachment to cholesterol is given. BODIPY-cholesterol became commercially available from Avanti Polar Lipids under the name “Top-Fluor” cholesterol from 2009 onwards.
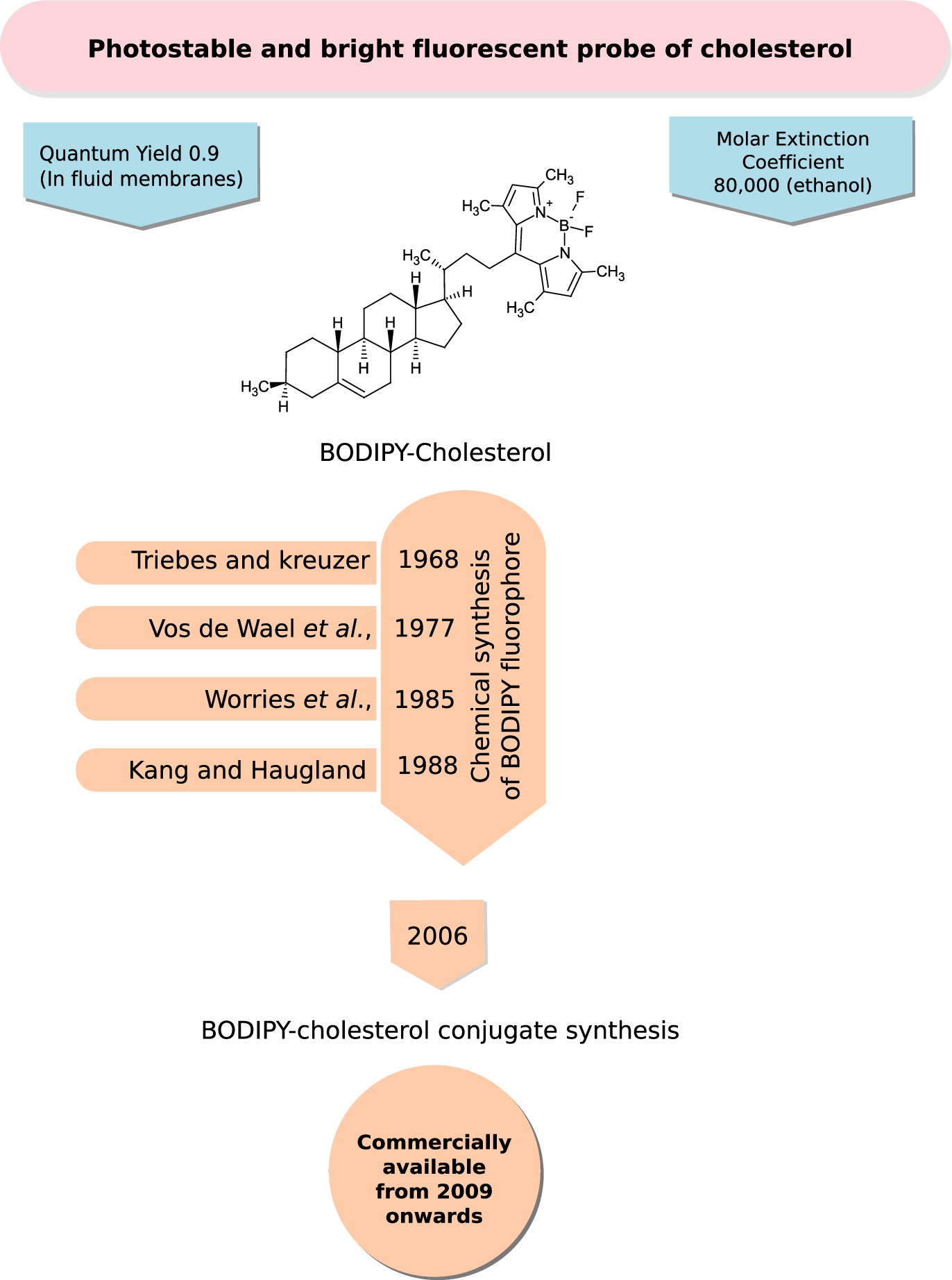
2.2.3.Alkyne cholesterol
Classic click reactions comprise a copper-catalyzed Huisgen azide–alkyne cycloaddition to form a substituted 1,2,3-triazole ring to label and detect molecules of interest in cells or tissues [46,72]. Recently, such clickable probes of cholesterol, alkyne cholesterol, were developed in Ned Porter’s laboratory in 2013 [169]; Lars Kuerschner’s group in 2014 [58] and Dai and Cheng’s laboratory in 2015 [80]. The difference between these three alkyne cholesterol analogs lies in the length of the alkyl chain, the one developed in Lars Kuerschner’s group preserves the native alkyl chain length. The longer alkyl chain alkyne cholesterol analogs [58,169] mimic native sterols in cellular studies with respect to both distribution and susceptibility to enzymatic reaction [58,169]. Alkyne cholesterol with the alkyl chain length introduced by Lars Kuerschner’s group became commercially available from Avanti Polar Lipids from 2015 onwards (personal communication with Dr. Stephen Burgess, Avanti Polar Lipids). Figure 6 gives the chemical structure of the commercially available form of alkyne cholesterol. A spectrum of azide-linked fluorescent probes are commercially available (Thermofisher Scientific, Jena Biosciences), which can be clicked to alkyne cholesterol (proprietary synthesis from Avanti Polar Lipids, see Fig. 6 for details). The applications of this sterol analog has been covered in details in recent reviews [118,154]. The limitation with this technique is its non-applicability to living cells owing to the harmful effect of Cu (I) and Cu (II), produced in presence of TCEP, on living cells.
Fig. 6.
Clickable analog of cholesterol, alkyne cholesterol. Click chemistry, especially the Cu(I)-catalyzed azide–alkyne cycloaddition, has provided membrane researchers with a palate of fluorophores, with different photo-physical properties, to label their biomolecule of interest. Three different forms of alkyne cholesterol (differing in the alkyl chain length) were synthesized between 2013 and 2015, and listed as a chronological timeline. The figure shows the chemical structure of the commercially available form of alkyne cholesterol introduced by Avanti Polar Lipids from 2015 onwards. The figure also provides a list of commercially available fluorophores (having different spectral and photo-physical properties), available from Thermofisher Scientific and Jena Biosciences, which can be clicked to alkyne cholesterol.
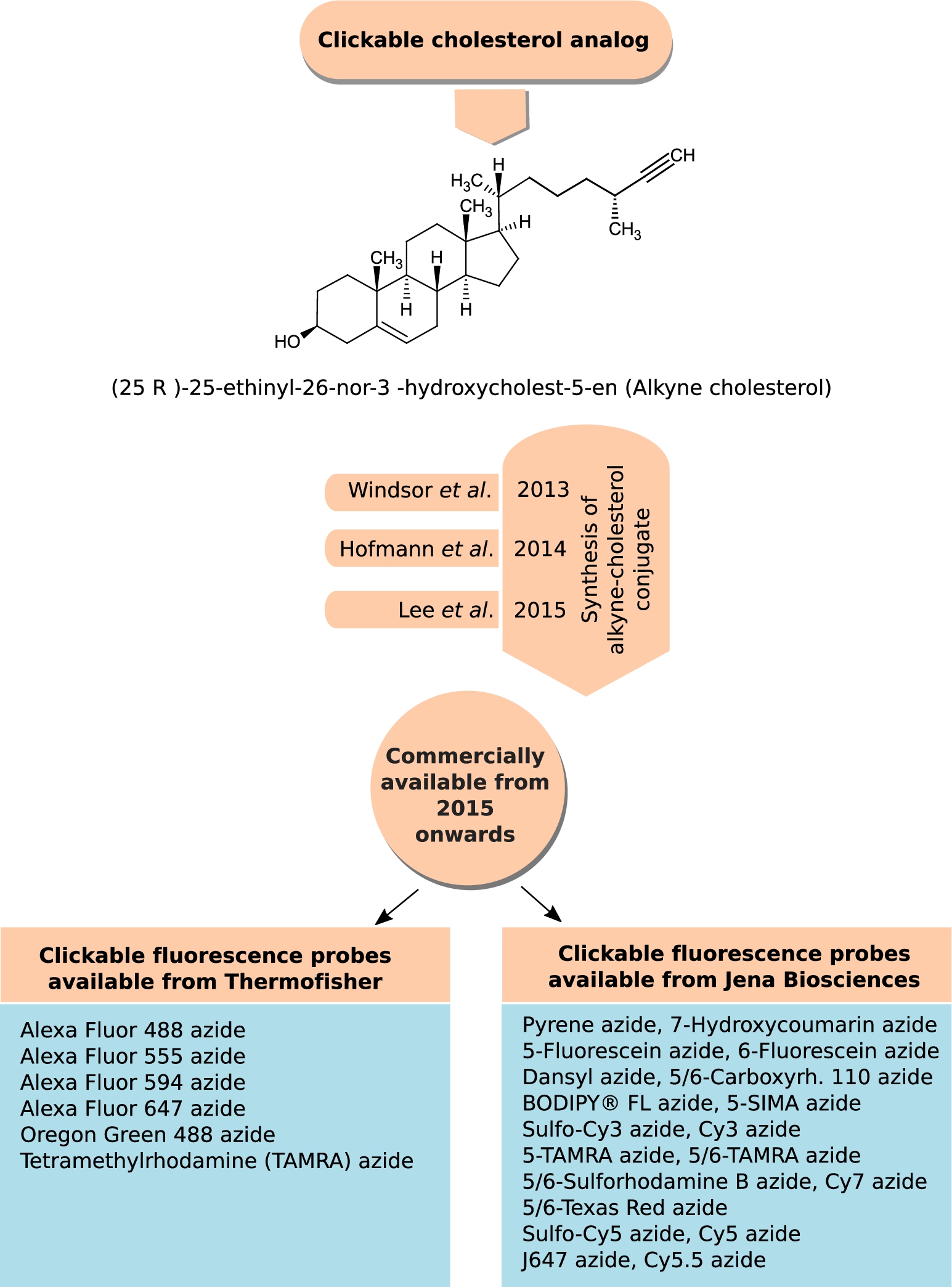
2.3.Toxins and polyene macrolide antibiotic binding to cholesterol: Perfringolysin O and filipin
Another class of molecules that has been used to probe cholesterol include the protein (toxin) Perfringolysin O (also known as θ-toxin) and the fluorescent polyene macrolide antibiotic filipin. Figure 7 maps the evolution of these molecules from their discovery to their present application as cholesterol bio-sensors.
Fig. 7.
Naturally occurring cytolysin (Perfringolysin O, PFO) and polyene macrolide antibiotic (filipin) binding to cholesterol. Perfringolysin O (a) and filipin (b) are the two naturally occurring biomolecules capable of binding cholesterol. PFO is produced by the pathogenic bacteria Clostridium perfringens, which is a leading cause of food poising [22]. Filipin is produced from Streptomyces filipinensis (originally isolated from soils of Philippines). The figure gives a chronological timeline describing their discovery and characterization of their mode of action. PFO was bio-engineered to reduce its cytotoxicity and cater to live-cell imaging as a cholesterol bio-sensor. Filipin staining remains a popular choice for labeling un-esterified cholesterol in fixed samples.
![Naturally occurring cytolysin (Perfringolysin O, PFO) and polyene macrolide antibiotic (filipin) binding to cholesterol. Perfringolysin O (a) and filipin (b) are the two naturally occurring biomolecules capable of binding cholesterol. PFO is produced by the pathogenic bacteria Clostridium perfringens, which is a leading cause of food poising [22]. Filipin is produced from Streptomyces filipinensis (originally isolated from soils of Philippines). The figure gives a chronological timeline describing their discovery and characterization of their mode of action. PFO was bio-engineered to reduce its cytotoxicity and cater to live-cell imaging as a cholesterol bio-sensor. Filipin staining remains a popular choice for labeling un-esterified cholesterol in fixed samples.](https://ip.ios.semcs.net:443/media/bsi/2017/6-1-2/bsi-6-1-2-bsi166/bsi-6-bsi166-g007.jpg)
Perfringolysin O, produced by Clostridium perfringens, belongs to a large family of cholesterol-binding/dependent cytolysins which also include other members such as streptolysin O produced by Streptococcus pyogenes and alveolysin by Bacillus alvei [77]. The toxin was first discovered by Herter around 1906 and was later described in detail in 1923 by Wuth [3]. By 1980’s, θ-toxin was shown to have a cholesterol dependent mode of action by studies on erythrocytes [106,160].
θ-toxin oligomerizes in the presence of cholesterol on cell membranes leading to pore formation and cell lysis. To decrease its cytotoxicity and extend its application to live-cell imaging, the toxin was bioengineered to produce non-cytolysing derivatives which could be fluorescently labelled, namely: (i) a biotinylated derivative BCθ [66], (ii) the D4 domain, which is the smallest functional unit comprising the cholesterol binding domain of θ-toxin [147] and (iii) a plasmid-based biosensor of cholesterol called D4H [93]. Fluorescently labelled avidin has been used to probe plasma-membrane cholesterol bound by BCθ [114,119]. Recombinant D4-fusion protein tagged with EGFP [65,147], mCherry [21,91] and Dronpa [107] have been utilized to visualize cholesterol in live cells. The recently introduced plasmid-based biosensor of cholesterol expresses cytosolic mCherry-tagged D4 with a mutation (D434S) that increases its affinity toward cholesterol. This was shown to bind to cholesterol in the cytoplasmic leaflet, relative to the other two variants (BCθ and D4) which don’t penetrate the cell and only bind the outer leaflet cholesterol of plasma membrane [90,98,114].
Filipin, a polyene antibiotic that forms a fluorescent complex with cholesterol, is commonly used to visualize the cellular distribution of free cholesterol [48,49]. Filipin was first isolated in 1955 from the mycelium and culture filtrates of actinomycete, Streptomyces jilipinensis, found in soil samples from Philipines [166]. Filipin is an antibiotic with antifungal properties and is a mixture of four macrolides with minor differences in their structure, the fraction known as filipin III being the major (
Filipin has been a popular fluorescent probe for monitoring the distribution of free non-esterified cholesterol from 1970’s onwards after Shroeder et al. [140] and Bittman and Fischkoff [12,13] showed that excitation and emission spectrum of filipin shows an increase in intensity but not in wavelength upon sterol binding. Despite its popularity as a cholesterol probe, it suffers from some limitations. Filipin is a UV-excitable dye and can be photo-bleached, making it difficult to obtain high-quality images [26,92,171]. In addition, it can be used only for fixed samples precluding it from live-cell imaging application. In addition in 2011, it was found that filipin was also labeling the GM1 ganglioside along with cholesterol [7]. Application of filipin in clinical research will be discussed later (see Section 2.3).
3.Application of fluorescent probes of cholesterol in clinical research
Research spanning over several decades have provided a wealth of information on the role of cholesterol in health and diseases. Molecular details of cholesterol-dependent mode of action of many important proteins (such as GPCRs and ion-channels) have been uncovered by an integration of biochemistry, biophysics and computational work and has been extensively covered in recent reviews [48,142]. However in this review, we largely confine the application of fluorescent analogs of cholesterol in clinical research, taking atherosclerosis, Niemann–Pick C and Alzheimer’s disease as representative examples (see Fig. 8 for a summary of the different fluorescent analogs of cholesterol applied for research and diagnosis of the three diseases).
Fig. 8.
Application of fluorescent probes of cholesterol in disease research. Cholesterol is a major lipid in eukaryotic membranes and has been associated with a plethora of diseases. The figure gives a summary of the fluorescent probes that have been used for research and diagnosis of the three representative diseases (atherosclerosis, Alzheimer’s and Niemann–Pick C disease) discussed in the review.
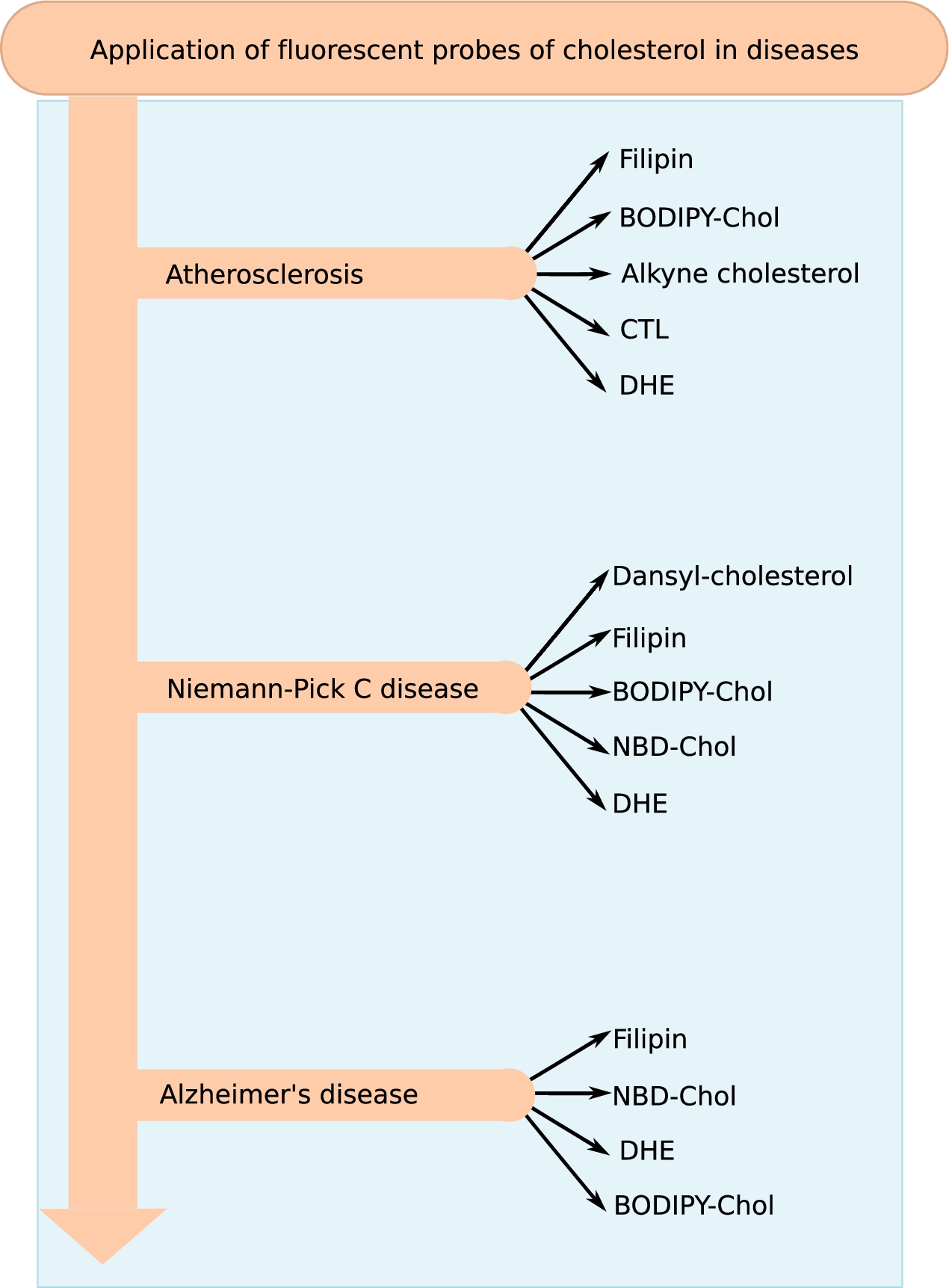
3.1.Athersoclerosis
Early lines of evidence linking hypercholesterolemia in humans to the progression of atherosclerosis and the risk of coronary heart disease have been reviewed robustly by others [156]. By the 1970’s, accumulated evidences motivated the search for interventions toward lowering blood cholesterol levels as a combinatorial therapy for atherosclerosis [157]. By the early 1980’s, the lipoprotein fractions LDL and HDL were known to be involved in the transport and metabolism of cholesterol, however, the precise structure of these particles and the mechanism by which they transport cholesterol between tissues remained unclear [75]. A direct consequence of this realization were the efforts launched toward the feeding of various animal models with fluorescent cholesterol and the histochemical analysis of tissues extracted from atherosclerotic lesions in these animal models [39,104,174].
Filipin has been a popular choice of probe from 1980’s onwards till date for histochemical detection and differentiation of free unesterified cholesterol. Filipin has been used for staining atherosclerotic lesions in experimentally induced rabbit and swine animal models [73,75]; hyperglycemic mouse model [15,165]; association of cholesterol accumulation with calcification in human atherosclerotic lesions [14,57]; and detection of cholesterol accumulation in lysosomes of macrophages in CD38−/− mice model [176]. In addition to filipin, CTL has also been fed to animal model of atherosclerosis to explore the thermotropic behavior of the sterol loaded LDL and HDL [9].
Over the years the understanding of cholesterol trafficking and homeostatis, orchestrated through action of various lipoproteins, has been a key focus to combat the development and progression of atherosclerosis [87]. Removal of excess cholesterol from peripheral tissues (cholesterol efflux) by HDL and delivery to the liver and steroidogenic tissues is an important pathway to remove excess cholesterol from circulating in blood and arrest the development of atherosclerosis [38]. NBD-cholesterol has been utilized to monitor the uptake of cholesterol [44,85] and its oxidized products [42] by HDL; and in assays for screening inhibitors for enzymes (ACAT-1 and ACAT-2) that performs intracellular cholesterol esterification, a process that is involved in development of atherosclerosis [79]. DHE and alkyne analog of cholesterol have been used to monitor the transfer of cholesterol via HDL [44,179]. In addition, using DHE it was shown that loading of free cholesterol does not trigger phase separation of the fluorescent sterol in the plasma membrane of macrophages [172]. BODIPY-cholesterol has also proven to be a probe of choice in assays measuring cholesterol efflux [97,138,146], screening inhibitors of enzymes (cholesteryl ester transfer protein) that catalyzes the exchange of cholesteryl ester and triglyceride between HDL and apo B containing lipoprotein particles [20].
Another important medical intervention to prevent atherosclerosis is lowering uptake and absorption of cholesterol from intestinal walls and keeping it in the intestinal lumen for excretion. Niemann–Pick C1-like 1 (NPC1L1) is a protein that highly expresses on the surface of the absorptive jejunal enterocytes and is responsible cholesterol uptake and absorption from intestine and is the drug target of ezetimibe [32]. A combination of CTL and DHE was used to understanding the effect of plasma-membranes cholesterol content on trafficking kinetics of NPC1L1, responsible for uptake and absorption of cholesterol from small intestine, between plasma membrane and recycling endosomes in hepatoma cells [127]. In a related study to explore the effect of dietary cholesterol on trafficking of intestinal NPC1L1 from the brush border to endosomes, uptake of NBD-cholesterol into explants from porcine jejunum was monitored [151].
3.2.Niemann–Pick C disease
Niemann–Pick type C (NPC) is a rare (
Filipin has been one of the earliest fluorescent probe to be used in investigations of cholesterol accumulation in lysosomes and around the Golgi region in mutant Type C Niemann–Pick fibroblasts derived from superficial skin biopsies of patients [143,153,162]. The decade of 1990’s saw the identification of homozygous mutations in either the NPC1 gene (responsible for
DHE has been used as a probe of choice in many studies exploring the structure-function relationship of NPC proteins in cholesterol trafficking. Cholesterol was shown to binding with sub-micromolar affinity to human NPC2 [43] and in 1:1 stoichiometry [88] using DHE as a fluorescent analog. Another interesting study using model membranes explored the cholesterol binding affinity and inter-membrane sterol transfer rates of wild type and mutant NPC2 protein using DHE fluorescence [101]. Kinetics of trafficking of sterols from plasma-membrane was explored in NPC cells using DHE and shown to accumulate in lysosomes as seen for LDL-derived sterol pool [129]. In another biophysical study, DHE was used as cholesterol mimetic to understand cholesterol transport in the presence of cyclodextrins, which belong to a group of cyclic oligosaccharides that bind to cholesterol and are routinely used for loading and depleting cholesterol from membranes [100]. Another interesting study using NBD-cholesterol in NPC fibroblasts showed that inhibition of cAMP reduced the load of cholesterol accumulation in these cells [95]. Cholesterol efflux in other models of NPC, such as NPC1-deficient CHO cells (M12 cells) [59] and human embryonic stem cells with NPC1 knockdown (KD) [130], were monitored using the bright and photostable analog of cholesterol, BODIPY-cholesterol. Dansyl-cholesterol has also been shown to be a good mimic in monitoring the trafficking of cholesterol in human NPC fibroblasts and other NPC-like cells [167].
3.3.Alzheimer’s disease (AD)
AD is a neurodegenerative disorder characterized by progressive and irreversible memory impairments, associated with cognitive decline and is a major cause of dementia and memory loss in the elderly population globally [45,53]. Increasing evidence in the last two decades have indicated neuronal cholesterol homeostasis to be an important factor in the pathogenesis of AD. These studies have demonstrated that cholesterol is increased in the frontal gyrus of AD patients and there is a progressive increase in cholesterol levels throughout the course of manifestation of the clinical disease [31,175]. A recent study showed that cholesterol efflux was altered in mild AD patients compared to the healthy subjects [19].
In view of the intricate role of cholesterol in the progression of AD, fluorescent probes of cholesterol have been used in exploring various tenets of the interplay of cholesterol with different biomolecular players. An example of this is a study where filipin staining of AD brains showed accumulation of cholesterol in congophilic/birefringent dense cores of senile plaques with respect to normal brains [111]. Additionally, the study showed a similar pattern of abnormal cholesterol accumulation in mature, congophilic amyloid plaques at 24 months of age in transgenic mice that overexpress the “Swedish” amyloid precursor protein (Tg APPsw, line 2576) [110]. Aggregation of the amyloid-beta (
4.Conclusion and future perspectives
Michel Chevreul was born in 1786 in Angers (France) and died as a centenarian at the age of 103 [81]. Chevruel was one of the pioneers in the study of various greasy material such as oils, butters, fat etc. In addition to naming cholesterol, Chevruel richly contributed to coining names of fats obtained from other sources such as “butyric” (for fatty acid obtained from butter) [81]. The legacy of Micheal Chevruel’s discovery of “cholesterol” as a non-saponifiable lipid from gall stones has ignited the imagination and research of many noble minds for over twenty decades now. In this review, we attempt to give a chronological account of some of the important developments which shaped the course of cholesterol research. We have focused on applications and research and advances in fluorescent analogs of cholesterol in exploring the missing links tagging cholesterol to various diseases. Fluorescence techniques have evolved over time to be more powerful in gleaning information from live-cells. With the advent of super-resolution microscopy techniques such as photoactivatable localization microscopy (PALM), direct stochastic optical reconstruction microscopy (dSTORM), and DNA-point accumulation for imaging in nanoscale topograph (PAINT), it has been possible to resolve sub-micron scale molecular interactions with precise molecule location co-ordinates. These insights could be used to quantitatively evaluate lipid-protein interaction through the determination of useful parameters such as density of bound conjugates, cluster size, and number of molecules per cluster [54]. Super-resolution imaging of interacting partners of cholesterol becomes relevant in the context of the identification of around 250 cholesterol-binding proteins (including receptors, ion-channels and enzymes) in a proteome-wide screen mapping the cholesterol-interacting proteins in mammalian cells [62].
Acknowledgements
Ar.C. gratefully acknowledges the support from Fulbright-Nehru post-doctoral fellowship. D.A. thanks Max-Planck-Institut für terrestrische Mikrobiologie for the award of a post-doctoral fellowship. We thank Catherine Deatherage for helpful comments and suggestion in preparation of the manuscript. We thank Andreas Max Ernst for his critical inputs on various aspects of lipid-protein interaction and for being an active member of the late night brain teasing sessions. We thank Stephen W. Burgess from Avanti Polar Lipids for his inputs on preparation of various timelines included in the review. Ar.C. takes this opportunity to thank Prof. Amitabha Chattopadhyay for introducing her to the world of cholesterol and membrane biology.
References
[1] | M.R. Alecio et al., Use of a fluorescent cholesterol derivative to measure lateral mobility of cholesterol in membranes, Proc. Natl. Acad. Sci. USA 79: ((1982) ), 5171–5174. doi:10.1073/pnas.79.17.5171. |
[2] | P.F.F. Almeida, Thermodynamics of lipid interactions in complex bilayers, Biochim. Biophys. Acta 1788: ((2009) ), 72–85. doi:10.1016/j.bbamem.2008.08.007. |
[3] | J.E. Alouf et al., Repertoire and general features of the family of cholesterol-dependent cytolysins, in: The Comprehensive Sourcebook of Bacterial Protein Toxins, 3rd edn, J.E. Alouf and M.R. Popoff, eds, Academic Press, Oxford, England, (2006) , pp. 643–658. doi:10.1016/B978-012088445-2/50041-X. |
[4] | R. Antonucci et al., |
[5] | D.B. Archer, The use of a fluorescent sterol to investigate the mode of action of amphotericin methyl ester, a polyene antibiotic, Biochem Biophys Res. Commun. 66: ((1975) ), 195–201. doi:10.1016/S0006-291X(75)80313-5. |
[6] | V. Aries and B.H. Kiksoi, Sterol biosynthesis by strains of Saccharomyces cerevisiae in the presence and absence of dissolved oxygen, J. Inst. Brew. 84: ((1978) ), 118–122. doi:10.1002/j.2050-0416.1978.tb03852.x. |
[7] | J.R. Arthur et al., Filipin recognizes both GM1 and cholesterol in GM1 gangliosidosis mouse brain, J. Lipid Res. 52: ((2011) ), 1345–1351. doi:10.1194/jlr.M012633. |
[8] | N.A. Avdulov et al., Lipid binding to amyloid beta-peptide aggregates: Preferential binding of cholesterol as compared with phosphatidylcholine and fatty acids, J. Neurochem. 69: ((1997) ), 1746–1752. doi:10.1046/j.1471-4159.1997.69041746.x. |
[9] | R. Bergeron and J. Scott, Fluorescent lipoprotein probe, Anal Biochem. 119: ((1982) ), 128–134. doi:10.1016/0003-2697(82)90675-3. |
[10] | W. Bergmann and P.G. Stevens, Studies on the conversion of ergosterol to adrenal cortical hormones, J. Org. Chem. 13: ((1948) ), 10–20. doi:10.1021/jo01159a002. |
[11] | C.E. Bills, Physiology of the sterols, including vitamin, Physiol. Rev. 15: ((1935) ), 1–97. |
[12] | R. Bittman et al., Interaction of filipin 3 and amphotericin B with lecithin-sterol vesicles and cellular membranes. Spectral and electron microscope studies, Biochemistry 13: ((1974) ), 1364–1373. doi:10.1021/bi00704a009. |
[13] | R. Bittman and S.A. Fischkoff, Fluorescence studies of the binding of the polyene antibiotics filipin III, amphotericin B, nystatin, and lagosin to cholesterol, Proc. Natl. Acad. Sci. USA 69: ((1972) ), 3795–3799. doi:10.1073/pnas.69.12.3795. |
[14] | Y.V. Bobryshev, Calcification of elastic fibers in human atherosclerotic plaque, Atherosclerosis 180: ((2005) ), 293–303. doi:10.1016/j.atherosclerosis.2005.01.024. |
[15] | A.J. Bowes, Valproate attenuates accelerated atherosclerosis in hyperglycemic apoE-deficient mice: Evidence in support of a role for endoplasmic reticulum stress and glycogen synthase kinase-3 in lesion development and hepatic steatosis, Am. J. Pathol. 174: ((2009) ), 330–342. doi:10.2353/ajpath.2009.080385. |
[16] | R.O. Brady et al., The metabolism of sphingomyelin. II. Evidence of an enzymatic deficiency in Niemann–Pick disease, Proc. Natl. Acad. Sci. USA 55: ((1966) ), 366–369. doi:10.1073/pnas.55.2.366. |
[17] | M.S. Brown and J.L. Goldstein, A receptor-mediated pathway for cholesterol homeostasis, Science, 232: ((1986) ), 34–47. |
[18] | P. Butko et al., Acidic phospholipids strikingly potentiate sterol carrier protein 2 mediated intermembrane sterol transfer, Biochemistry 29: ((1990) ), 4070–4077. doi:10.1021/bi00469a007. |
[19] | P. Camponova et al., Alteration of high-density lipoproteins functionality in Alzheimer’s disease patients, Can J Physiol Pharmacol ((2017) ) 2017 Apr 6 [Epub ahead of print]. doi:10.1139/cjpp-2016-0710. |
[20] | G. Cao et al., Evacetrapib is a novel, potent, and selective inhibitor of cholesteryl ester transfer protein that elevates HDL cholesterol without inducing aldosterone or increasing blood pressure, J Lipid Res 52: ((2011) ), 2169–2176. doi:10.1194/jlr.M018069. |
[21] | M. Carquin et al., Cholesterol segregates into submicrometric domains at the living erythrocyte membrane: Evidence and regulation, Cell Mol. Life Sci. 72: ((2015) ), 4633–4651. doi:10.1007/s00018-015-1951-x. |
[22] | A. Charlebois et al., Tolerance of Clostridium perfringens biofilms to disinfectants commonly used in the food industry, Food Microbiol. 62: ((2017) ), 32–38. doi:10.1016/j.fm.2016.09.009. |
[23] | A. Chattopadhyay, Chemistry and biology of N-(7-nitrobenz-2-oxa-1, 3-diazol-4-yl)-labeled lipids: Fluorescent probes of biological and model membranes, Chem. Phys. Lipids 53: ((1990) ), 1–15. doi:10.1016/0009-3084(90)90128-E. |
[24] | A. Chattopadhyay and E. London, Parallax method for direct measurement of membrane penetration depth utilizing fluorescence quenching by spin labeled phospholipids, Biochemistry 26: ((1987) ), 39–45. doi:10.1021/bi00375a006. |
[25] | A. Chattopadhyay and E. London, Spectroscopic and ionization properties of N (7 nitro 2, 1,3 benzoxadiazol 4 yl) labeled lipids in model membranes, Biochim. Biophys. Acta 938: ((1988) ), 24–34. doi:10.1016/0005-2736(88)90118-6. |
[26] | A. Chattopadhyay, S. Shrivastava and A. Chaudhuri, Membrane fluorescent probes: Insights and perspectives, in: Fluorescent Analogues of Biomolecular Building Blocks: Design and Applications, Y. Tor and M. Wilhelmsson, eds, John Wiley, (2016) , pp. 356–366. doi:10.1002/9781119179320.ch15. |
[27] | R.F. Chen, Fluorescence of dansyl amino acids in organic solvents and protein solutions, Arch Biochem Biophys 120: ((1967) ), 609–626. doi:10.1016/0003-9861(67)90526-7. |
[28] | V. Cherezov et al., High-resolution crystal structure of an engineered human |
[29] | P.L. Chong and T.E. Thompson, Depolarization of dehydroergosterol in phospholipid bilayers, Biochim. Biophys. Acta 863: ((1986) ), 53–62. doi:10.1016/0005-2736(86)90386-X. |
[30] | A.T. Clay et al., Interaction of the P-glycoprotein multidrug transporter with sterols, Biochemistry 54: ((2015) ), 6586–6597. doi:10.1021/acs.biochem.5b00904. |
[31] | R.G. Cutler et al., Involvement of oxidative stress-induced abnormalities in ceramide and cholesterol metabolism in brain aging and Alzheimer’s disease, Proc. Natl. Acad. Sci. USA 101: ((2004) ), 2070–2075. doi:10.1073/pnas.0305799101. |
[32] | H.R. Davis and E.P. Veltri, Zetia: Inhibition of Niemann–Pick C1 like 1 (NPC1L1) to reduce intestinal cholesterol absorption and treat hyperlipidemia, J. Atheroscler. Thromb. 14: ((2007) ), 99–108. doi:10.5551/jat.14.99. |
[33] | R.F.M. de Almeida et al., Cholesterol modulates the organization of the gammaM4 transmembrane domain of the muscle nicotinic acetylcholine receptor, Biophys. J. 86: ((2004) ), 2261–2272. doi:10.1016/S0006-3495(04)74284-8. |
[34] | B. de Kruijff et al., Polyene antibiotic-sterol interactions in membranes of Acholeplasma laidlawii cells and lecithin liposomes. I. Specificity of the membrane permeability changes induced by the polyene antibiotics, Biochim Biophys Acta 339: ((1974) ), 30–43. doi:10.1016/0005-2736(74)90330-7. |
[35] | V. de Wael et al., Pyrromethene-BF, complexes (4,4′-difluoro-4-bora-3a,4a-diaza-s-indacenes). Synthesis and luminescence properties, Recl. Trav. Chim. Pays-Bas 96: ((1977) ), 306–309. |
[36] | C. Delseth et al., Ergosta-5,7,9(11), 22-tetraen-3β-ol and its 24ϵ-ethyl homolog, two new marine sterols from the Red Sea sponge biemna fortis, Helv. Chim. Acta 62: ((1979) ), 2037–2045. doi:10.1002/hlca.19790620633. |
[37] | W.R.H. Evans and C.J. Hendriks, Niemann–Pick type C disease – the tip of the iceberg? A review of neuropsychiatric presentation, diagnosis and treatment, BJPsych Bull. 41: ((2017) ), 109–114. |
[38] | E. Favari et al., Cholesterol efflux and reverse cholesterol transport, Handb Exp Pharmacol 224: ((2015) ), 181–206. doi:10.1007/978-3-319-09665-0_4. |
[39] | G. Finking and H. Hanke, Nikolaj Nikolajewitsch Anitschkow (1885–1964) established the cholesterol-fed rabbit as a model for atherosclerosis research, Atherosclerosis 135: ((1997) ), 1–7. doi:10.1016/S0021-9150(97)00161-5. |
[40] | R.T. Fischer et al., Structure and dynamic properties of dehydroergosterol, |
[41] | R.T. Fischer et al., Fluorescence of delta 5, 7,9(11), 22-ergostatetraen-3 beta-ol in micelles, sterol carrier protein complexes, and plasma membranes, Biochemistry 24: ((1985) ), 3322–3331. doi:10.1021/bi00334a037. |
[42] | K. Fluiter et al., Scavenger receptor BI mediates the selective uptake of oxidized cholesterol esters by rat liver, J. Biol. Chem. 274: ((1999) ), 8893–8899. doi:10.1074/jbc.274.13.8893. |
[43] | N. Friedland et al., Structure of a cholesterol-binding protein deficient in Niemann–Pick type C2 disease, Proc. Natl. Acad. Sci. USA 100: ((2003) ), 2512–2517. doi:10.1073/pnas.0437840100. |
[44] | A. Frolov, High density lipoprotein-mediated cholesterol uptake and targeting to lipid droplets in intact L-cell fibroblasts. A single- and multiphoton fluorescence approach, J. Biol. Chem. 275: ((2000) ), 12769–12780. doi:10.1074/jbc.275.17.12769. |
[45] | Y. Fu et al., ABCA5 regulates amyloid-β peptide production and is associated with Alzheimer’s disease neuropathology, J. Alzheimers Dis. 43: ((2015) ), 857–869. |
[46] | A. Gaebler et al., Alkyne lipids as substrates for click chemistry-based in vitro enzymatic assays, J. Lipid Res. 54: ((2013) ), 2282–2290. doi:10.1194/jlr.D038653. |
[47] | P.B. Ghosh and M.W. Whitehouse, 7-chloro-4-nitrobenzo-2-oxa-1, 3-diazole: A new fluorigenic reagent for amino acids and other amines, Biochem. J. 108: ((1968) ), 155–156. doi:10.1042/bj1080155. |
[48] | G. Gimpl, Cholesterol-protein interaction: Methods and cholesterol reporter molecules, Subcell Biochem. 51: ((2010) ), 1–45. doi:10.1007/978-90-481-8622-8_1. |
[49] | G. Gimpl and K. Gehrig-Burger, Cholesterol reporter molecules, Biosci. Rep. 27: ((2007) ), 335–358. doi:10.1007/s10540-007-9060-1. |
[50] | D. Gottlieb, H.E. Carter, J.H. Sioneker and A. Ammann, Protection of fungi against polyene antibiotics by sterols, Science 128: ((1958) ), 361. doi:10.1126/science.128.3320.361. |
[51] | Z. Guo et al., Recent progress in the development of near-infrared fluorescent probes for bioimaging applications, Chem. Soc. Rev. 43: ((2014) ), 16–29. doi:10.1039/C3CS60271K. |
[52] | S. Haldar and A. Chattopadhyay, Application of NBD-labeled lipids in membrane and cell biology, in: Springer Series on Fluorescence, Vol. 13: , Y. Mely and G. Duportail, eds, Springer, Heidelberg, (2013) , pp. 37–50. |
[53] | S. Hannaoui et al., Cholesterol balance in prion diseases and Alzheimer’s disease, Viruses 6: ((2014) ), 4505–4535. doi:10.3390/v6114505. |
[54] | J.M. Hartley et al., Super-resolution imaging and quantitative analysis of membrane protein/lipid raft clustering mediated by cell-surface self-assembly of hybrid nanoconjugates, Chembiochem. 16: ((2015) ), 1725–1729. doi:10.1002/cbic.201500278. |
[55] | K. Harzer and B. Kustermann-Kuhn, Quantified increases of cholesterol, total lipid and globotriaosylceramide in filipin-positive Niemann–Pick type C fibroblasts, Clin. Chim Acta 305: ((2001) ), 65–73. doi:10.1016/S0009-8981(00)00421-6. |
[56] | R.P. Haugland and H.C. Kang, Chemically reactive dipyrrometeneboron difluoride dyes; US. Patent 4, 774 (1988) 339. |
[57] | D. Hirsch et al., Colocalization of cholesterol and hydroxyapatite in human atherosclerotic lesions, Calcif. Tissue Int. 52: ((1993) ), 94–98. doi:10.1007/BF00308315. |
[58] | K. Hofmann et al., A novel alkyne cholesterol to trace cellular cholesterol metabolism and localization, J. Lipid Res. 55: ((2014) ), 583–591. doi:10.1194/jlr.D044727. |
[59] | M. Hölttä-Vuori et al., BODIPY-cholesterol: A new tool to visualize sterol trafficking in living cells and organisms, Traffic 9: ((2008) ), 1839–1849. |
[60] | R.W. Holz, Polyene antibiotics: Nystatin, amphotericin B, and filipin, in: Antibiotics V-2, F.E. Hahn, ed., Springer-Verlag, New York, (1979) , pp. 313–340. |
[61] | H. Huang et al., Use of dansyl-cholestanol as a probe of cholesterol behavior in membranes of living cells, J. Lipid Res. 51: ((2010) ), 1157–1172. doi:10.1194/jlr.M003244. |
[62] | J.J. Hulce et al., Proteome-wide mapping of cholesterol-interacting proteins in mammalian cells, Nat Methods 10: ((2013) ), 259–264. doi:10.1038/nmeth.2368. |
[63] | D.B. Iaea et al., Role of STARD4 in sterol transport between the endocytic recycling compartment and the plasma membrane, Mol Biol Cell ((2017) ). doi:10.1091/mbc.E16-07-0499. |
[64] | U. Igbavboa et al., Amyloid beta-protein stimulates trafficking of cholesterol and caveolin-1 from the plasma membrane to the Golgi complex in mouse primary astrocytes, Neuroscience 162: ((2009) ), 328–338. doi:10.1016/j.neuroscience.2009.04.049. |
[65] | R. Ishitsuka et al., Fluorescence image screening for chemical compounds modifying cholesterol metabolism and distribution, J. Lipid Res. 52: ((2011) ), 2084–2094. doi:10.1194/jlr.D018184. |
[66] | M. Iwamoto et al., A biotinylated perfringolysin O derivative: A new probe for detection of cell surface cholesterol, Biochim Biophys Acta 1327: ((1997) ), 222–230. doi:10.1016/S0005-2736(97)00061-8. |
[67] | Y.J. Kao et al., N-(2-naphthyl)-23, 24-dinor-5-cholen-22-amin-3beta-ol, a fluorescent cholesterol analogue, Biochemistry 17: ((1978) ), 2689–2696. doi:10.1021/bi00606a036. |
[68] | J. Karolin et al., Fluorescence and absorption spectroscopic properties of dipyrrometheneboron difluoride (BODIPY) derivatives in liquids, lipid membranes, and proteins, J. Am. Chem. Soc. 116: ((1994) ), 7801–7806. doi:10.1021/ja00096a042. |
[69] | R.A. Kenner and A.A. Aboderin, A new fluorescent probe for protein and nucleoprotein conformation. Binding of 7-(p-methoxybenzylamino)-4-nitrobenzoxadiazole to bovine trypsinogen and bacterial ribosomes, Biochemistry 10: ((1971) ), 4433–4440. doi:10.1021/bi00800a013. |
[70] | A.S. Klymchenko and R. Kreder, Fluorescent probes for lipid rafts: From model membranes to living cells, Chem. Biol. 21: ((2014) ), 97–113. doi:10.1016/j.chembiol.2013.11.009. |
[71] | M.H. Ko and L. Puglielli, The sterol carrier protein SCP-x/pro-SCP-2 gene has transcriptional activity and regulates the Alzheimer disease gamma-secretase, J. Biol. Chem. 282: ((2007) ), 19742–19752. doi:10.1074/jbc.M611426200. |
[72] | H.C. Kolb and K.B. Sharpless, The growing impact of click chemistry on drug discovery, Drug Discov. Today 8: ((2003) ), 1128–1137. doi:10.1016/S1359-6446(03)02933-7. |
[73] | H.S. Kruth, Histochemical detection of esterified cholesterol within human atherosclerotic lesions using the fluorescent probe filipin, Atherosclerosis 51: ((1984) ), 281. doi:10.1016/0021-9150(84)90175-8. |
[74] | H.S. Kruth et al., Type C Niemann–Pick disease. Abnormal metabolism of low density lipoprotein in homozygous and heterozygous fibroblasts, J. Biol. Chem. 261: ((1986) ), 16769–16774. |
[75] | H.S. Kruth and D.L. Fry, Histochemical detection and differentiation of free and esterified cholesterol in swine atherosclerosis using filipin, Exp. Mol. Pathol. 40: ((1984) ), 288–294. doi:10.1016/0014-4800(84)90046-7. |
[76] | E.M. Kuech et al., Alterations in membrane trafficking and pathophysiological implications in lysosomal storage disorders, Biochimie 130: ((2016) ), 152–162. doi:10.1016/j.biochi.2016.09.011. |
[77] | L. Kuerschner and C. Thiele, Multiple bonds for the lipid interest, Biochim. Biophys. Acta 1841: ((2014) ), 1031–1037. doi:10.1016/j.bbalip.2013.12.018. |
[78] | A. Kyrychenko, Using fluorescence for studies of biological membranes: A review, Methods Appl. Fluoresc. 3: ((2015) ), 042003. doi:10.1088/2050-6120/3/4/042003. |
[79] | A.T. Lada et al., Identification of ACAT1- and ACAT2-specific inhibitors using a novel, cell-based fluorescence assay: Individual ACAT uniqueness, J Lipid Res 45: ((2004) ), 378–386. doi:10.1194/jlr.D300037-JLR200. |
[80] | H.J. Lee et al., Assessing cholesterol storage in live cells and C. elegans by stimulated Raman scattering imaging of phenyl-diyne cholesterol, Sci. Rep. 5: ((2015) ), 7930. doi:10.1038/srep07930. |
[81] | P. Lemay and R.E. Oesper, Michel Eugene Chevreul (1786–1889), J. Chem. Educ. 25: ((1948) ), 62–70. doi:10.1021/ed025p62. |
[82] | J.J. Li, Triumph of the Heart: The Story of Statins, Oxford University Press, (2009) . |
[83] | Z. Li et al., First synthesis of free cholesterol-BODIPY conjugates, J. Org. Chem. 71: ((2006) ), 1718–1721. doi:10.1021/jo052029x. |
[84] | D.A. Lightner, Early scientific investigations, in: Bilirubin: Jekyll and Hyde Pigment of Life, Springer, Vienna, (2013) , pp. 9–175. doi:10.1007/978-3-7091-1637-1_2. |
[85] | H.Y. Lim et al., Lymphatic vessels are essential for the removal of cholesterol from peripheral tissues by SR-BI-mediated transport of HDL, Cell Metab. 17: ((2013) ), 671–684. doi:10.1016/j.cmet.2013.04.002. |
[86] | M.D. Linder et al., Rab8-dependent recycling promotes endosomal cholesterol removal in normal and sphingolipidosis cells, Mol. Biol. Cell. 18: ((2007) ), 47–56. doi:10.1091/mbc.E06-07-0575. |
[87] | M.F. Linton et al., SR-BI: A multifunctional receptor in cholesterol homeostasis and atherosclerosis, Trends Endocrinol Metab ((2017) ), pii: S1043-2760(17)30017-6. |
[88] | H.L. Liou et al., NPC2, the protein deficient in Niemann–Pick C2 disease, consists of multiple glycoforms that bind a variety of sterols, J. Biol. Chem. 281: ((2006) ), 36710–36723. doi:10.1074/jbc.M608743200. |
[89] | L. Liscum and K.W. Underwood, Intracellular cholesterol transport and compartmentation, J. Biol. Chem. 270: ((1995) ), 15443–15446. doi:10.1074/jbc.270.26.15443. |
[90] | M. Maekawa, Domain 4 (D4) of perfringolysin O to visualize cholesterol in cellular membranes – the update, Sensors (Basel) 17: ((2017) ), 3. |
[91] | M. Maekawa et al., Staurosporines decrease ORMDL proteins and enhance sphingomyelin synthesis resulting in depletion of plasmalemmal phosphatidylserine, Sci. Rep. 6: ((2016) ), 35762. doi:10.1038/srep35762. |
[92] | M. Maekawa and G.D. Fairn, Molecular probes to visualize the location, organization and dynamics of lipids, J Cell Sci 127: ((2014) ), 4801–4812. doi:10.1242/jcs.150524. |
[93] | M. Maekawa and G.D. Fairn, Complementary probes reveal that phosphatidylserine is required for the proper transbilayer distribution of cholesterol, J Cell Sci 128: ((2015) ), 1422–1433. doi:10.1242/jcs.164715. |
[94] | N. Mani, The historical background of clinical chemistry, J. Clin. Chem. Clin. Biochem. 19: ((1981) ), 311–322. |
[95] | M.E. Manson et al., cAMP-mediated regulation of cholesterol accumulation in cystic fibrosis and Niemann–Pick type C cells, Am J Physiol Lung Cell Mol Physiol 295: ((2008) ), L809–L819. doi:10.1152/ajplung.90402.2008. |
[96] | D.L. Marks et al., Use of bodipy-labeled sphingolipid and cholesterol analogs to examine membrane microdomains in cells, Histochem. Cell Biol. 130: ((2008) ), 819–832. doi:10.1007/s00418-008-0509-5. |
[97] | C. Martel et al., Lymphatic vasculature mediates macrophage reverse cholesterol transport in mice, J. Clin. Invest. 123: ((2013) ), 1571–1579. doi:10.1172/JCI63685. |
[98] | M. Masashi et al., Perfringolysin O theta toxin as a tool to monitor the distribution and inhomogeneity of cholesterol in cellular membranes, Toxins (Basel) 8: ((2016) ), 67. doi:10.3390/toxins8030067. |
[99] | F.R. Maxfield and D. Wüstner, Analysis of cholesterol trafficking with fluorescent probes, Methods Cell Biol. 108: ((2012) ), 367–393. doi:10.1016/B978-0-12-386487-1.00017-1. |
[100] | L.A. McCauliff et al., Sterol transfer between cyclodextrin and membranes: Similar but not identical mechanism to NPC2-mediated cholesterol transfer, Biochemistry 50: ((2011) ), 7341–7349. doi:10.1021/bi200574f. |
[101] | L.A. McCauliff et al., Multiple surface regions on the Niemann–Pick C2 protein facilitate intracellular cholesterol transport, J. Biol. Chem. 290: ((2015) ), 27321–27331. doi:10.1074/jbc.M115.667469. |
[102] | A.L. McIntosh et al., Fluorescent sterols for the study of cholesterol trafficking in living cells, in: Probes and Tags to Study Biomolecular Function: For Proteins, RNA, and Membranes, L.W. Miller, ed., Wiley-VCH Verlag GmbH & Co. KGaA, Weinheim, Germany, (2008) . |
[103] | A.L. McIntosh et al., Fluorescence techniques using dehydroergosterol to study cholesterol trafficking, Lipids 43: ((2008) ), 1185–1208. doi:10.1007/s11745-008-3194-1. |
[104] | K.S. Meir and E. Leitersdorf, Atherosclerosis in the apolipoprotein-E-deficient mouse: A decade of progress, Arterioscler. Thromb. Vasc. Biol. 24: ((2004) ), 1006–1014. doi:10.1161/01.ATV.0000128849.12617.f4. |
[105] | T. Meyer et al., Membrane properties of cholesterol analogs with an unbranched aliphatic side chain, Chem. Phys. Lipids 184: ((2014) ), 1–6. doi:10.1016/j.chemphyslip.2014.08.002. |
[106] | K. Mitsui et al., Effects of cholesterol evulsion on susceptibility to perfringolysin O of human erythrocytes, Biochim. Biophys. Acta 686: ((1982) ), 177–181. doi:10.1016/0005-2736(82)90110-9. |
[107] | H. Mizuno et al., Fluorescent probes for super-resolution imaging of lipid domains on the plasma membrane, Chem. Sci. 2: ((2011) ), 1538–1553. |
[108] | M. Modzel et al., Synthesis and live-cell imaging of fluorescent sterols for analysis of intracellular cholesterol transport, Methods Mol. Biol. 1583: ((2017) ), 111–140. doi:10.1007/978-1-4939-6875-6_10. |
[109] | M. Mondal et al., Sterols are mainly in the cytoplasmic leaflet of the plasma membrane and the endocytic recycling compartment in CHO cells, Mol. Biol. Cell. 20: ((2009) ), 581–588. doi:10.1091/mbc.E08-07-0785. |
[110] | I. Morgado and M. Garvey, Lipids in amyloid-β processing, aggregation, and toxicity, Adv Exp Med Biol 855: ((2015) ), 67–94. doi:10.1007/978-3-319-17344-3_3. |
[111] | T. Mori et al., Cholesterol accumulates in senile plaques of Alzheimer disease patients and in transgenic APP(SW) mice, J. Neuropathol. Exp. Neurol. 60: ((2001) ), 778–785. doi:10.1093/jnen/60.8.778. |
[112] | O.G. Mouritsen and M.J. Zuckermann, What’s so special about cholesterol?, Lipids 39: ((2004) ), 1101–1113. doi:10.1007/s11745-004-1336-x. |
[113] | P. Nair, Brown and Goldstein: The cholesterol chronicles, Proc. Natl. Acad. Sci. USA 110: ((2013) ), 14829–14832. doi:10.1073/pnas.1315180110. |
[114] | M. Nakamura et al., Cellular aging-dependent decrease in cholesterol in membrane microdomains of human diploid fibroblasts, Exp. Cell Res. 290: ((2003) ), 381–390. doi:10.1016/S0014-4827(03)00343-4. |
[115] | T. Nakamura et al., Phosphatidylcholine liposomes containing cholesterol analogues with side chains of various lengths, Chem. Phys. Lipids 26: ((1980) ), 101–110. doi:10.1016/0009-3084(80)90014-6. |
[116] | A.W. Norman et al., Studies on the biological properties of polyene antibiotics. Evidence for the direct interaction of filipin with cholesterol, J. Biol. Chem. 247: ((1972) ), 1918–1929. |
[117] | A.W. Norman et al., Studies on the biological properties of polyene antibiotics: Comparison of other polyenes with filipin in their ability to interact specifically with sterol, Biochim Biophys Acta 290: ((1972) ), 1–14. doi:10.1016/0005-2736(72)90046-6. |
[118] | Y. Ohno-Iwashita et al., Perfringolysin O, a cholesterol-binding cytolysin, as a probe for lipid rafts, Anaerobe 10: ((2004) ), 125–134. doi:10.1016/j.anaerobe.2003.09.003. |
[119] | Y. Ohno-Iwashita et al., Cholesterol-binding toxins and anti-cholesterol antibodies as structural probes for cholesterol localization, Subcell Biochem. 51: ((2010) ), 597–621. doi:10.1007/978-90-481-8622-8_22. |
[120] | H. Osman et al., Mediation of elicitin activity on tobacco is assumed by elicitin-sterol complexes, Mol. Biol. Cell. 12: ((2001) ), 2825–2834. doi:10.1091/mbc.12.9.2825. |
[121] | R.C. Pandey and K.L. Rinehart, Polyene antibiotics. V. Characterization of components of the filipin complex, J. Antibiot. 23: ((1970) ), 414–417. doi:10.7164/antibiotics.23.414. |
[122] | M. Pasenkiewicz-Gierula et al., Cholesterol effects on the phosphatidylcholine bilayer polar region: A molecular simulation study, Biophys. J. 78: ((2000) ), 1376–1389. doi:10.1016/S0006-3495(00)76691-4. |
[123] | M.C. Patterson and S.U. Walkley, Niemann–Pick disease, type C and Roscoe Brady, Mol Genet Metab. 120: ((2017) ), 34–37. doi:10.1016/j.ymgme.2016.11.008. |
[124] | K.B. Peake and J.E. Vance, Defective cholesterol trafficking in Niemann–Pick C-deficient cells, FEBS Lett. 584: ((2010) ), 2731–2739. doi:10.1016/j.febslet.2010.04.047. |
[125] | P.G. Pentchev et al., A genetic storage disorder in BALB/C mice with a metabolic block in esterification of exogenous cholesterol, J. Biol. Chem. 259: ((1984) ), 5784–5791. |
[126] | P.G. Pentchev et al., The cholesterol storage disorder of the mutant BALB/c mouse. A primary genetic lesion closely linked to defective esterification of exogenously derived cholesterol and its relationship to human type C Niemann–Pick disease, J. Biol. Chem. 261: ((1986) ), 2772–2777. |
[127] | N.H. Petersen et al., Kinetic imaging of NPC1L1 and sterol trafficking between plasma membrane and recycling endosomes in hepatoma cells, J. Lipid Res. 49: ((2008) ), 2023–2037. doi:10.1194/jlr.M800145-JLR200. |
[128] | A.D. Petrescu et al., Fluorescent sterols monitor cell penetrating peptide Pep-1 mediated uptake and intracellular targeting of cargo protein in living cells, Biochim Biophys Acta 1788: ((2009) ), 425–441. doi:10.1016/j.bbamem.2008.09.015. |
[129] | N.H. Pipalia et al., Sterol, protein and lipid trafficking in Chinese hamster ovary cells with Niemann–Pick type C1 defect, Traffic 8: ((2007) ), 130–141. doi:10.1111/j.1600-0854.2006.00513.x. |
[130] | W.C. Plaisted et al., Disruption and therapeutic rescue of autophagy in a human neuronal model of Niemann Pick type C1, Hum. Mol. Genet. 21: ((2012) ), 2651–2662. doi:10.1093/hmg/dds090. |
[131] | L. Pokhrel et al., Inhibition of Acyl-CoA: Cholesterol acyltransferase (ACAT), overexpression of cholesterol transporter gene, and protection of amyloid β ( |
[132] | L. Qiu et al., Cholesterol modulates the interaction of beta-amyloid peptide with lipid bilayers, Biophys. J. 96: ((2009) ), 4299–4307. doi:10.1016/j.bpj.2009.02.036. |
[133] | H. Raghuraman and A. Chattopadhyay, Interaction of melittin with membrane cholesterol: A fluorescence approach, Biophys. J. 87: ((2004) ), 2419–2432. doi:10.1529/biophysj.104.043596. |
[134] | L. Rajendran et al., Efficient inhibition of the Alzheimer’s disease beta-secretase by membrane targeting, Science 320: ((2008) ), 520–523. doi:10.1126/science.1156609. |
[135] | D.M.C. Ramirez et al., NBD-cholesterol probes to track cholesterol distribution in model membranes, Biochim. Biophys. Acta 1798: ((2010) ), 558–568. doi:10.1016/j.bbamem.2009.12.005. |
[136] | J. Rogers et al., The organisation of cholesterol and ergosterol in lipid bilayers based on studies using non-perturbing fluorescent sterol probes, Biochim. Biophys. Acta 552: ((1979) ), 23–37. doi:10.1016/0005-2736(79)90243-8. |
[137] | W.V. Ruyle et al., The preparation of |
[138] | S. Sankaranarayanan et al., A sensitive assay for ABCA1-mediated cholesterol efflux using BODIPY-cholesterol, J Lipid Res 52: ((2011) ), 2332–2340. doi:10.1194/jlr.D018051. |
[139] | H.A. Scheidt et al., Cholesterol’s aliphatic side chain modulates membrane properties, Angew Chem. Int Ed Engl. 52: ((2013) ), 12848–12851. doi:10.1002/anie.201306753. |
[140] | F. Schroeder et al., Fluorometric evidence for the binding of cholesterol to the filipin complex, J. Antibiot. 24: ((1971) ), 846–849. doi:10.7164/antibiotics.24.846. |
[141] | F. Schroeder et al., Sterol and squalene carrier protein interactions with fluorescent delta 5,7,9(11)-cholestatrien-3 beta-ol, J. Biol. Chem. 260: ((1985) ), 2904–2911. |
[142] | D. Sengupta et al., Interaction of membrane cholesterol with GPCRs: Implications in receptor oligomerization, in: G Protein-Coupled Receptor Dimers, G. Giovanni, K. Herrick-Davis and G. Milligan, eds, Springer, Heidelberg (in press). |
[143] | N.J. Severs, Cholesterol cytochemistry in cell biology and disease, Subcell Biochem. 28: ((1997) ), 477–505. doi:10.1007/978-1-4615-5901-6_16. |
[144] | E. Sezgin et al., Partitioning, diffusion, and ligand binding of raft lipid analogs in model and cellular plasma membranes, Biochim Biophys Acta 1818: ((2012) ), 1777–1784. doi:10.1016/j.bbamem.2012.03.007. |
[145] | E. Sezgin et al., A comparative study on fluorescent cholesterol analogs as versatile cellular reporters, J. Lipid Res. 57: ((2016) ), 299–309. |
[146] | J.A. Shaw et al., Cholesterol efflux in megakaryocyte progenitors suppresses platelet production and thrombocytosis, Nat. Med. 19: ((2013) ), 586–594. doi:10.1038/nm.3150. |
[147] | Y. Shimada et al., The C-terminal domain of perfringolysin O is an essential cholesterol binding unit targeting to cholesterol-rich microdomains, Eur. J. Biochem 269: ((2002) ), 6195–6203. doi:10.1046/j.1432-1033.2002.03338.x. |
[148] | S. Shrivastava et al., Orientation and dynamics of a novel fluorescent cholesterol analogue in membranes of varying phase, J. Phys. Chem. B 113: ((2009) ), 4475–4481. doi:10.1021/jp808309u. |
[149] | D. Sica et al., Sterols of Candida tropicalis grown on n-alkanes, Phytochemistry 21: ((1982) ), 234–236. doi:10.1016/0031-9422(82)80056-3. |
[150] | K. Simons and E. Ikonen, How cells handle cholesterol, Science 290: ((2000) ), 1721–1726. doi:10.1126/science.290.5497.1721. |
[151] | M. Skov et al., Dietary cholesterol induces trafficking of intestinal Niemann–Pick type C1 like 1 from the brush border to endosomes, Am. J. Physiol. Gastrointest. Liver Physiol 300: ((2011) ), G33–G40. doi:10.1152/ajpgi.00344.2010. |
[152] | R.J.M. Smith and C. Green, Fluorescence studies of protein-sterol relationships in human plasma lipoproteins, Biochem. J. 137: ((1974) ), 413–415. doi:10.1042/bj1370413. |
[153] | J. Sokol et al., Type C Niemann–Pick disease. Lysosomal accumulation and defective intracellular mobilization of low density lipoprotein cholesterol, J. Biol. Chem. 263: ((1988) ), 3411–3417. |
[154] | K.A. Solanko et al., Fluorescent sterols and cholesteryl esters as probes for intracellular cholesterol transport, Lipid Insights 8: ((2015) ), 95–114. |
[155] | L.M. Solanko et al., Membrane orientation and lateral diffusion of BODIPY-cholesterol as a function of probe structure, Biophys. J. 105: ((2013) ), 2082–2092. doi:10.1016/j.bpj.2013.09.031. |
[156] | D. Steinberg, Thematic review series: The pathogenesis of atherosclerosis. An interpretive history of the cholesterol controversy: Part I, J. Lipid Res. 45: ((2004) ), 1583–1593. doi:10.1194/jlr.R400003-JLR200. |
[157] | D. Steinberg, Thematic review series: The pathogenesis of atherosclerosis. An interpretive history of the cholesterol controversy: Part II: The early evidence linking hypercholesterolemia to coronary disease in humans, J Lipid Res 46: ((2005) ), 179–190. doi:10.1194/jlr.R400012-JLR200. |
[158] | T. Terai and T. Nagano, Fluorescent probes for bioimaging applications, Curr. Opin. Chem. Biol. 12: ((2008) ), 515–521. doi:10.1016/j.cbpa.2008.08.007. |
[159] | A. Treibs and F.-H. Kreuzer, Difluorboryl-Komplexe von Di- und Tripyrrylmethenen, Liebigs Annalen Chem 718: ((1968) ), 208–223. |
[160] | R.K. Tweten, Cholesterol-dependent cytolysins, a family of versatile pore-forming toxins, Infect. Immun. 73: ((2005) ), 6199–6209. doi:10.1128/IAI.73.10.6199-6209.2005. |
[161] | J.E. Vance and B. Karten, Niemann–Pick C disease and mobilization of lysosomal cholesterol by cyclodextrin, J. Lipid Res. 55: ((2014) ), 1609–1621. doi:10.1194/jlr.R047837. |
[162] | M.T. Vanier et al., Type C Niemann–Pick disease: Spectrum of phenotypic variation in disruption of intracellular LDL-derived cholesterol processing, Biochim. Biophys. Acta 1096: ((1991) ), 328–337. doi:10.1016/0925-4439(91)90069-L. |
[163] | G. Weber, Polarization of the fluorescence of macromolecules. 2. Fluorescent conjugates of ovalbumin and bovine serum albumin, Biochem. J. 51: ((1952) ), 155–167. doi:10.1042/bj0510155. |
[164] | M.M. Weber and S.C. Kinsky, Effect of cholesterol on the sensitivity of Mycoplasma laidlawii to the polyene antibiotic filipin, J. Bacteriol. 89: ((1965) ), 306–312. |
[165] | G.H. Werstuck, Glucosamine-induced endoplasmic reticulum dysfunction is associated with accelerated atherosclerosis in a hyperglycemic mouse model, Diabetes 55: ((2006) ), 93–101. doi:10.2337/diabetes.55.01.06.db05-0633. |
[166] | G.B. Whitfield et al., Filipin, an antifungal antibiotic: Isolation and properties, J. Am. Chem. Soc. 77: ((1955) ), 4799–4801. doi:10.1021/ja01623a032. |
[167] | V. Wiegand et al., Transport of plasma membrane-derived cholesterol and the function of Niemann–Pick C1 protein, FASEB J. 17: ((2003) ), 782–784. |
[168] | A. Windaus and O. Linsert, Ober die Eltraviolett-Bestrahlung des Dehydro-ergosterins, Justus Liebigs Ann. Chem. 465: ((1928) ), 148–166. doi:10.1002/jlac.19284650108. |
[169] | K. Windsor et al., Probing lipid-protein adduction with alkynyl surrogates: Application to Smith–Lemli–Opitz syndrome, J. Lipid Res. 54: ((2013) ), 2842–2850. doi:10.1194/jlr.M041061. |
[170] | H.J. Wories et al., A novel water-soluble fluorescent probe: Synthesis, luminescence and biological properties of the sodium salt of the 4-sulfonato-3,3′,5,5′-tetramethyl-2,2′-pyrromethen-l,l′-BF, complex, Recl. Trav. Chim. Pays-Bas 104: ((1985) ), 288. doi:10.1002/recl.19851041104. |
[171] | D. Wüstner, Fluorescent sterols as tools in membrane biophysics and cell biology, Chem. Phys. Lipids 146: ((2007) ), 1–25. doi:10.1016/j.chemphyslip.2006.12.004. |
[172] | D. Wüstner, Free-cholesterol loading does not trigger phase separation of the fluorescent sterol dehydroergosterol in the plasma membrane of macrophages, Chem Phys Lipids 154: ((2008) ), 129–136. doi:10.1016/j.chemphyslip.2008.04.009. |
[173] | D. Wüstner et al., Quantitative assessment of sterol traffic in living cells by dual labeling with dehydroergosterol and BODIPY-cholesterol, Chem. Phys. Lipids 164: ((2011) ), 221–235. doi:10.1016/j.chemphyslip.2011.01.004. |
[174] | L. Xiangdong et al., Animal models for the atherosclerosis research: A review, Protein Cell 2: ((2011) ), 189–201. doi:10.1007/s13238-011-1016-3. |
[175] | H. Xiong et al., Cholesterol retention in Alzheimer’s brain is responsible for high beta- and gamma-secretase activities and A-beta production, Neurobiol. Dis. 29: ((2008) ), 422–437. doi:10.1016/j.nbd.2007.10.005. |
[176] | X. Xu et al., Lysosomal cholesterol accumulation in macrophages leading to coronary atherosclerosis in CD38(-/-) mice, J Cell Mol Med 20: ((2016) ), 1001–1013. doi:10.1111/jcmm.12788. |
[177] | Z. Xu et al., Regulation of sterol transport between membranes and NPC2, Biochemistry 47: ((2008) ), 11134–11143. doi:10.1021/bi801328u. |
[178] | J. Yguerabide and M.C. Foster, Fluorescence spectroscopy of biological membranes membrane spectroscopy, in: Molecular Biology Biochemistry and Biophysics, Vol. 31: , E. Grell, ed., Springer, Berlin, (1981) , pp. 199–269. |
[179] | J. Zhang et al., Development of a cell-based, high-throughput screening assay for cholesterol efflux using a fluorescent mimic of cholesterol, Assay Drug Dev. Technol. 9: ((2011) ), 136–146. doi:10.1089/adt.2010.0288. |