Serotonergic Regulation of Synaptic Dopamine Levels Mitigates L-DOPA-Induced Dyskinesia in a Mouse Model of Parkinson’s Disease
Abstract
Background:
The serotonin (5-HT) system can manipulate the processing of exogenous L-DOPA in the DA-denervated striatum, resulting in the modulation of L-DOPA-induced dyskinesia (LID).
Objective:
To characterize the effects of the serotonin precursor 5-hydroxy-tryptophan (5-HTP) or the serotonin transporter (SERT) inhibitor, Citalopram on L-DOPA-induced behavior, neurochemical signals, and underlying protein expressions in an animal model of Parkinson’s disease.
Methods:
MitoPark (MP) mice at 20 weeks of age, subjected to a 14-day administration of L-DOPA/Carbidopa, displayed dyskinesia, referred to as LID. Subsequent investigations explored the effects of 5-HT-modifying agents, such as 5-HTP and Citalopram, on abnormal involuntary movements (AIMs), locomotor activity, neurochemical signals, serotonin transporter activity, and protein expression in the DA-denervated striatum of LID MP mice.
Results:
5-HTP exhibited duration-dependent suppressive effects on developing and established LID, especially related to abnormal limb movements observed in L-DOPA-primed MP mice. However, Citalopram, predominantly suppressed abnormal axial movement induced by L-DOPA in LID MP mice. We demonstrated that 5-HTP could decrease L-DOPA-upregulation of DA turnover rates while concurrently upregulating 5-HT metabolism. Additionally, 5-HTP was shown to reduce the expressions of p-ERK and p-DARPP-32 in the striatum of LID MP mice. The effect of Citalopram in alleviating LID development may be attributed to downregulation of SERT activity in the dorsal striatum of LID MP mice.
Conclusions:
While both single injection of 5-HTP and Citalopram effectively mitigated the development of LID, the difference in mitigation of AIM subtypes may be linked to the unique effects of these two serotonergic agents on L-DOPA-derived DA and 5-HT metabolism.
INTRODUCTION
Levodopa (L-DOPA) replacement is the primary intervention for Parkinson’s disease (PD). However, protracted daily exposure over time may lead to motor response fluctuations and abnormal involuntary movements (AIMs), known as levodopa-induced dyskinesia (LID) [1, 2]. Despite the intricate and not fully elucidated nature of the mechanisms underlying LID, its fundamental origins are linked to alterations in L-DOPA-derived dopamine (DA) metabolism, resulting in the hyperactivation of striatal DA receptors and associated signaling pathways [3–7].
L-DOPA crosses the blood-brain barrier (BBB) and, following metabolic conversion via aromatic L-amino acid decarboxylase (AADC), generates DA. Subsequently, DA is sequestered by the vesicular monoamine transporter 2 (VMAT2) into synaptic vesicles and is ultimately released into the synaptic cleft [8]. While dopaminergic neurons are primarily responsible for DA synthesis, storage and secretion, serotonergic neurons also contain enzymes like AADC and VMAT2, suggesting their potential involvement in DA production. Therefore, some studies proposed that under conditions of dopamine neuronal damage, serotonin neurons may absorb exogenous L-DOPA and, through intraneuronal AADC and VMAT2, synthesize and release DA. However, the serotonin system lacks mechanisms regulating DA release, leading to an excess of synaptic DA and the onset of LID [9]. In rodents with impaired dopamine neurons, prolonged L-DOPA treatment increases the density of serotonergic neuronal fibers in the striatal region. Concurrently, an upregulation in AADC expression is evident, indicating adaptive changes in serotonin neurons following extended L-DOPA exposure [10]. These investigations highlight the involvement of the serotonin system in the context of LID. Consequently, researchers have increasingly considered that serotonin system function may have a mitigating effect on LID.
Numerous findings from both preclinical and clinical research have emphasized the efficacy of modulating the serotonin system in preventing and treating LID [11] [12]. This includes promoting 5-HT1A or 5-HT1B receptors [13–15] and inhibiting 5-HT2A receptors [16] or the serotonin transporter (SERT) [17, 18]. Unfortunately, certain drugs directly targeting serotonin receptors have been shown to induce adverse effects during clinical trials, such as exacerbating PD symptoms [19, 20] and causing a 5-HT syndrome [21]. However, SERT inhibitors appear to have milder side effects in treating LID, compared to drugs that regulate serotonin receptors [21]. While the underlying causes of these side effects remain unclear, several studies proposed that this is related to these drugs interfering with the interaction between DA and serotonin [11, 22]. To alleviate the side effects of serotonin therapy in LID treatment, some studies have chosen the direct administration of the serotonin precursor 5-hydroxytryptophan (5-HTP) owing to its beneficial characteristics. Unlike serotonin, 5-HTP can easily penetrate the blood-brain barrier [23]. Additionally, it regulates excessive DA levels without affecting serotonin levels, unlike serotonin autoreceptor modulators. Furthermore, 5-HTP treatment was shown to exert an anti-dyskinetic effect in an LID rat model without compromising the efficacy of L-DOPA [24]. Importantly, a study by Meloni et al. involving seven LID patients who received 50 mg of 5-HTP with L-DOPA for 4 weeks showed significant improvements in dyskinesia compared to the placebo group [25]. Despite these promising findings, a comprehensive understanding of how 5-HTP or SERT inhibitors modulate serotonin systems in response to various excitatory signals in LID remains unclear. Additional preclinical research is required to elucidate the neurochemical mechanisms underlying the alleviation of LID development by serotoninergic drugs. This could contribute to the formulation of novel strategies for serotonin therapy in treating LID [11].
This study aimed to investigate the role of the serotonin system on DA release and abnormal involuntary movements in L-DOPA-responsive LID mice. Our LID animal model was established by continuously administering L-DOPA to MitoPark mice for 14 days, as shown in previous research [26]. Subsequently, two distinct treatment regimens were conducted. Prior to L-DOPA administration in LID MP mice, either the serotonin precursor, 5-HTP, or the SERT inhibitor, Citalopram, was administered to modulate DA dynamics in both the presynaptic neurons and the synaptic cleft. Through neurochemical and cellular analyses, we explored the effects of these serotonergic target-specific modulators on monoamine neurotransmission and dyskinesia-related biochemical markers in LID mice. This study is the first to elucidate the role of serotonin systems in the development of LID by examining the correlation between monoamine neurotransmission and abnormal involuntary movements in L-DOPA-responsive LID mice.
MATERIALS AND METHODS
Animals
Following NIH guidelines, approval for all animal protocols was secured from the National Defense Medical Center (NDMC) Animal Care and Use Committee (IACUC 22-008 and IACUC 23-207). Detailed information about experimental protocols and the timing of drug administration are provided in Figs. 1, 2A, 3A, and 5A.
Fig. 1
The experimental protocol for this study involves categorizing the experimental mice into four groups: normal animals (WT), PD mice (MP), MP mice with L-DOPA-induced dyskinesia (MP LID), and the MP LID treatment group. L-DOPA and Carbidopa were administered daily to the MP LID mice from Day 1 to Day 6, while WT and MP mice received only L-DOPA and Carbidopa from Day 3 to Day 6. The experiment commenced after administering L-DOPA and Carbidopa from Day 3 to Day 5. On Day 6, 10 min after administering L-DOPA and Carbidopa, the mice were euthanized by cervical dislocation, and their brains were then removed for subsequent experiments.
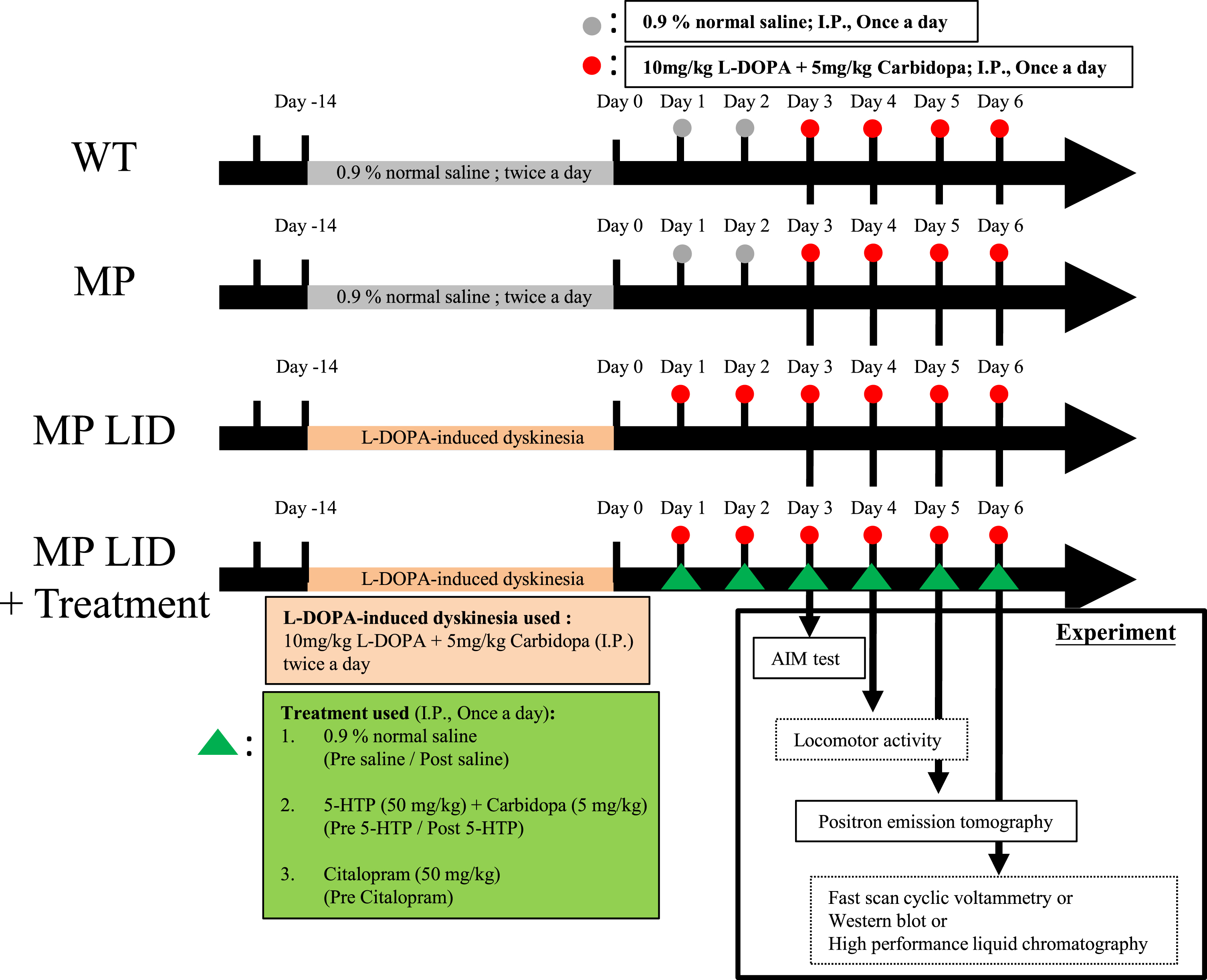
Fig. 2
Administering 5-HTP (50 mg/kg) 30 min before L-DOPA (10 mg/kg) treatment was an effective method for mitigating the abnormal AIM scores induced by L-DOPA in L-DOPA-primed MP mice (MP LID mice). A) The influence of pre-treatment with 5-HTP at time intervals of 30, 60, 90, and 120 min before the administration of L-DOPA on the L-DOPA-induced dyskinesia in MP LID mice. B) MP LID mice were pre-administered 5-HTP, followed by the administration of L-DOPA at different time intervals. Following L-DOPA administration, AIM scores were assessed at 20-min intervals, with a total of seven evaluations. Two-way ANOVA followed by a Bonferroni post hoc test for multiple comparisons. At 30 min compared to baseline, *p < 0.05, ***p < 0.001; at 60 min compared to baseline, ##p < 0.01, ###p < 0.001. Observation of the seven scores separately across the time span of 20 to 140 min post-L-DOPA administration, (C) the maximum AIM score from the seven scores (maximum AIM score), and (D) the sum of the seven scores (total AIM score). The maximum AIM score and the total AIM score were both lower when L-DOPA was administered 30 min after pre-treatment with 5-HTP (represented by the blue bar/rectangular symbol; 30 min), as compared to the other pre-treatment 5-HTP groups and the group without 5-HTP pre-treatment (represented by the red bar/rectangular symbol; Baseline). In particular, the administration of L-DOPA 60 min after pre-treatment with 5-HTP (represented by the purple bar/triangle symbol; 60 min) resulted in an elevation in the total AIM score compared to the group without 5-HTP pre-treatment (Baseline vs. 60 min, p < 0.01). One-way ANOVA followed by a Bonferroni post hoc test for multiple comparisons. At 30 min compared to the other group, *p < 0.05, **p < 0.01, ***p < 0.001; at 60 min compared to Baseline, ##p < 0.01.
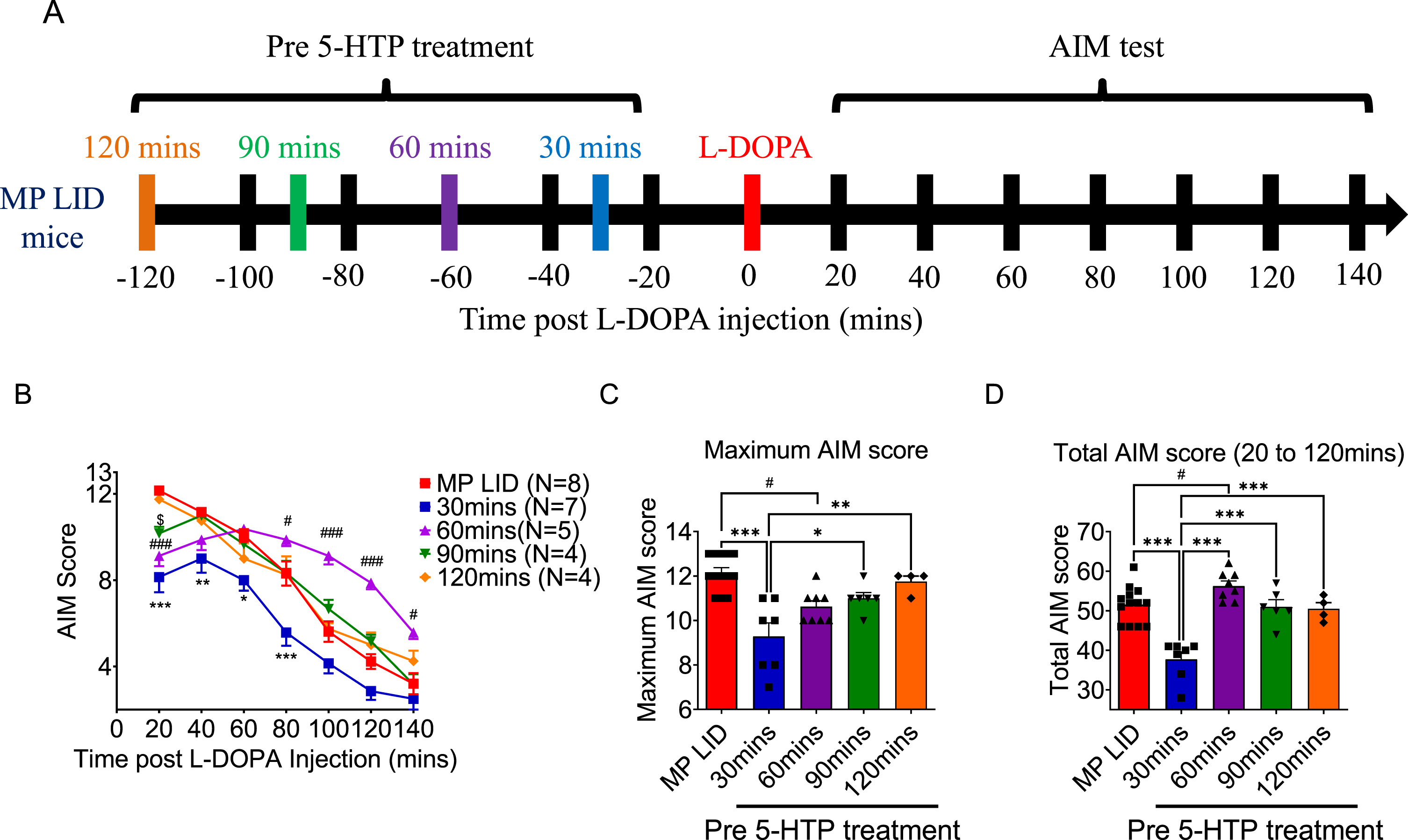
Fig. 3
Either pre-treatment or post-treatment with 5-HTP can effectively alleviate L-DOPA-induced dyskinesia in MP LID mice. A) The protocol for administering 5-HTP in MP LID mice involved the following steps: The assessment of AIM scores began immediately after the injection of L-DOPA and continued at 20-min intervals for a total duration of 120 min. B) Although dyskinesia in MP LID mice was found following administration of L-DOPA, the subsequent treatment with 5-HTP alleviated the abnormal symptoms in these mice. Following a 20-min administration of L-DOPA, post-treatment with 5-HTP reduces the abnormal AIM scores at the 80th and 100th min (Two-way ANOVA followed by a Bonferroni post hoc test for multiple comparisons. MP LID + L-DOPA + Saline compared to MP LID + 5-HTP + L-DOPA, ***p < 0.001), (C) as well as reduces the cumulative AIM score from the 20th to the 120th min. One-way ANOVA followed by a Bonferroni post hoc test for multiple comparisons. MP LID + L-DOPA + Saline compared to MP LID + 5-HTP + L-DOPA, ***p < 0.001. D) Locomotor analysis showed significant increases in MP LID groups after L-DOPA injection. The increase in vertical movement observed in the MP LID group following L-DOPA injection was mitigated by pre- or post-treatment with 5-HTP. (MP LID + L-DOPA + saline vs. MP LID + 5-HTP + L-DOPA, p < 0.001; MP LID + L-DOPA + saline vs. MP LID + L-DOPA + 5-HTP, p < 0.05). One-way ANOVA followed by a Bonferroni post hoc test for multiple comparisons. *p < 0.05, ***p < 0.001, compared to MP LID + L-DOPA + Saline; MP + L-DOPA compared to MP LID + L-DOPA + Saline, ###p < 0.001; WT compared to MP, $$$p < 0.001. E) The administration of 5-HTP as a pre-treatment or post-treatment did not result in a reduction of the increased horizontal movement observed after L-DOPA injection. One-way ANOVA followed by a Bonferroni post hoc test for multiple comparisons. MP + L-DOPA compared to MP LID + L-DOPA + Saline, ###p < 0.001; WT compared to MP, $$p < 0.01.
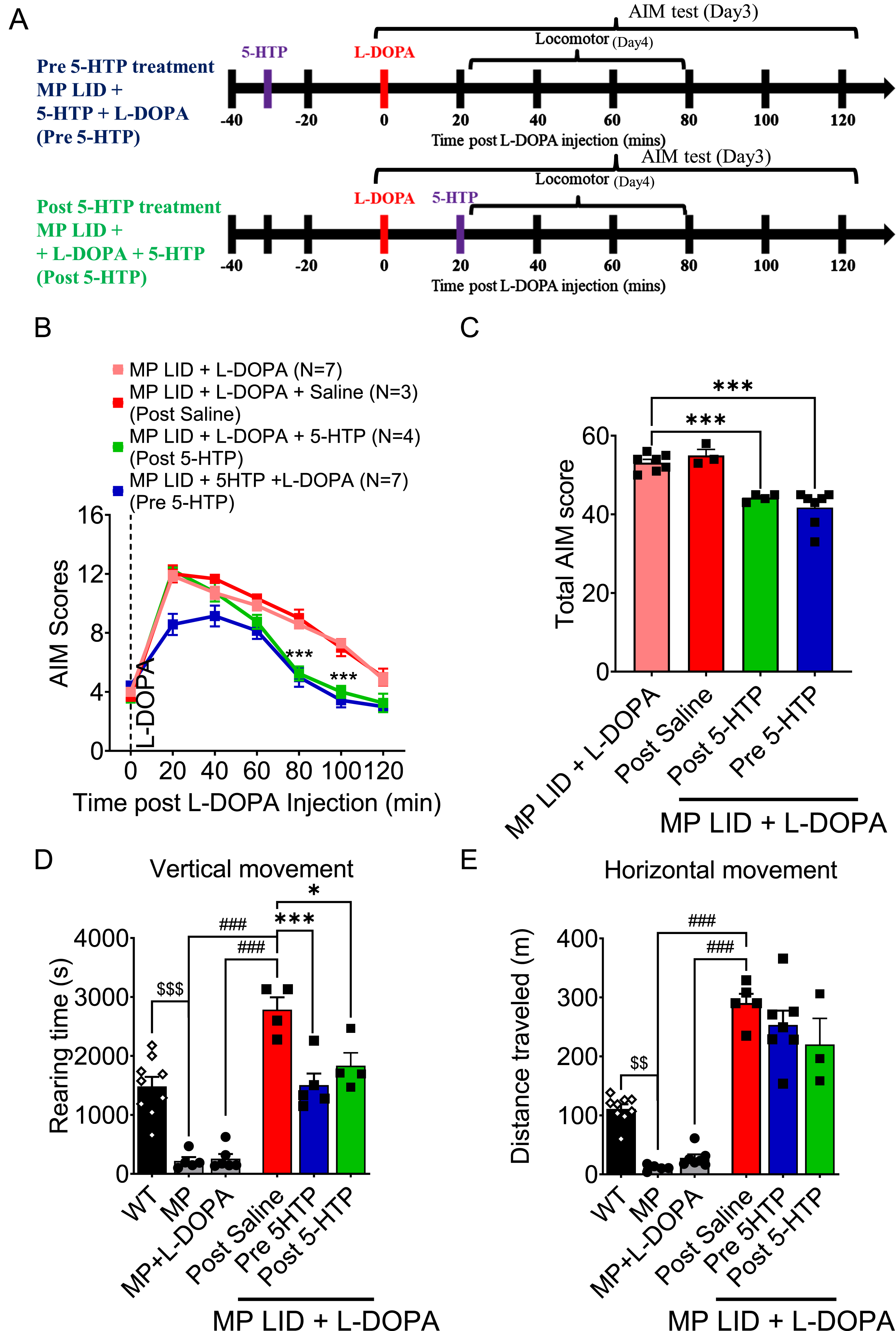
This experiment used 40 wild-type (WT) and 151 MitoPark (MP) mice, with 106 MP mice developing L-dopa-induced dyskinesia (LID). The experimental animals assigned to each experiment are detailed in Supplementary Table 1. This study utilized different individual experimental animals for in vitro experiments, including high performance liquid chromatography (HPLC), western blot (WB), and fast scan cyclic voltammetry (FSCV). The 20-week-old male mice were housed at 25°C with a 12/12 light/dark cycle and continuous water and food supply in the animal facility at NDMC with full AAALAC accreditation. The main aim of this study was to investigate the role of the serotonin system in LID. Previous research has found that estrogen affects the serotonin system [27, 28], PD symptoms [29] and LID [30]. In addition, PD symptoms may be influenced by menstrual cycles [31]. Hence, in these experiments, male mice were used to preclude the interference of estrogen.
The breeding strategy employed to generate MP mice has been detailed in previous publications [32–34]. The MP mice utilized in this series of experiments were heterozygous for DAT-cre expression (DAT/DATcre), and homozygous for the loxP-flanked Tfam gene (TfamloxP/TfamloxP). Breeding pairs used for the generation of MP mice were originally sourced from a colony maintained at the NIDA (NIH) Intramural Program. Subsequently, these MP mouse breeding pairs were sent to the NDMC in Taiwan, where comprehensive genotyping procedures were undertaken.
Drug administration
In this experiment, dyskinesia was induced in MP mice through the prolonged administration of L-DOPA and carbidopa (Sigma-Aldrich, St. Louis, MO, USA). L-DOPA (10 mg/kg)/carbidopa (5 mg/kg) was administered twice daily, in the morning and late afternoon (10 and 18 h within the 24-h clock), and initiated in the MP mice at the age of 20 weeks, continuing for 14 days. During the L-DOPA/carbidopa priming process, the brain undergoes sensitization, altering its response to subsequent dopaminergic therapies. Therefore, this experimental group is denoted by the symbol ‘MP LID’. We examined the involvement of the serotonergic system in L-DOPA-induced dyskinesia in MP LID mice by administering serotonergic drugs. All drugs utilized in this study were dissolved in 0.9% saline and delivered via intraperitoneal injection to the experimental animals.
The first objective of this study was to assess the influence of the serotonin precursor, 5-HTP, on L-DOPA-induced dyskinesia in MP LID mice. MP LID mice that were administered L-DOPA/carbidopa were subjected to either pre-treatment 30 min or post-treatment 20 min with 5-HTP (50 mg/kg)/carbidopa (5 mg/kg) or 0.9% saline, administered once daily for 6 consecutive days.
Subsequently, we also examined the effects of pre-treatment with the SERT inhibitor, Citalopram (50 mg/kg) on L-DOPA-induced dyskinesia in MP LID mice. MP LID mice received Citalopram 30 min before L-DOPA/carbidopa administration once daily for 6 consecutive days.
MP LID mice underwent daily experiments, beginning on the third day following the administration of 5-HTP or Citalopram. These experiments included the AIM test, and locomotor test, PET scans, and HPLC or FSCV, and were conducted sequentially.
Behavioral tests
AIM test. The four types of movements, Axial, Forelimb, Standing, and Paw movements, exhibited by the mice were captured using a webcam. Subsequently, the investigators utilized video recordings to assess the motor performance of the mice. We adhered to the modified AIM guidelines for the evaluation of abnormal involuntary movements, assigning scores on a scale from 0 to 4 [35, 36]. Axial displacement indicates the most significant deviation in mouse head/neck performance observed within a 1-min timeframe. Torsion of the neck/upper trunk by at least 30°, 60°, 90°, and >90° leads to mice losing their balance, correlating with 1, 2, 3, and 4 points, respectively. Forelimb movement of the mouse within a 1-min timeframe is observed, and corresponding scores are assigned. One point is awarded for a tiny displacement of the forepaw around a fixed position, while two points are assigned for large movements causing visible displacement of the entire forelimb, typically towards or away from the snout. Three points are given for significant displacement of the entire forelimb with visible engagement of shoulder muscles, and four points are awarded for vigorous limb displacement crossing over the midline of the body. Standing involves the continuous standing time of mice within 1 min. Continuous standing for 1 to 5, 6 to 10, 11 to 15, and exceeding 20 s represents scores of 1, 2, 3, and 4, respectively. Abnormal paw movements are scored by observing the performance of the mouse’s paw on the surface of the cylinder wall. Mice standing on both hind paws, with one or both front paws moving repeatedly up and down along the surface of the cylinder wall, are scored 1 and 2 points. Mice standing on their hind paws close to the wall of the cylinder and moving both front paws as described above, along with repeatedly lifting one or both hind paws up and down, score 3 and 4 points. Each of the four items are noted once every minute. The highest score achieved within 1 min based on the above grading is considered the score for this item. The AIM score is calculated as the sum of the four items within a 1-min timeframe (Supplementary Figure 1). Following the administration of L-DOPA/carbidopa to the experimental animals, assessments were conducted at 20-min intervals, resulting in a total of six evaluations. In order to conduct a thorough evaluation of drug effects on behavioral performance, the experiment illustrated in Fig. 2 was conducted seven times, with the remaining experiments evaluated six times. The scores obtained from these six or seven assessments were then aggregated to calculate the overall AIM score. A higher AIM score indicated more pronounced abnormal involuntary movement behaviors. In our experiment, the average total AIM score for normal mice (WT mice) remained below 30 points, whereas the total AIM score for MP LID mice with dyskinesia exceeded 30 points.
Locomotor activity. After the AIM test, the animals underwent a locomotor activity test on the following day. After allowing the mice to habituate in a low-noise environment for 1 h, we used activity boxes (45×45 cm) to evaluate horizontal and vertical movements. L-DOPA (10 mg/kg) and carbidopa (5 mg/kg) were administered to all experimental animals 10 min prior to conducting the locomotor activity testing. The protocol involved the use of an infrared beam grid to monitor mouse movements for 1 h, quantifying both the horizontal distance traveled and the frequency of rearing behaviors.
[18F] FE-ADAM positron emission tomography (PET) imaging of SERT function
Our previous paper provided details regarding the synthesis of [18F]FE-ADAM [37]. Mice received a tail vein injection of 0.3 mCi [18F]FE-ADAM while under anesthesia (5% isoflurane/oxygen mixture for induction, reduced to 2% for maintenance). Subsequently, all experimental animals were administered L-DOPA (10 mg/kg) and carbidopa (5 mg/kg). Following injection, the mice were placed inside a radiation barrier box. After a 20-min incubation period, the mice were positioned within the PET scanner for a static image scan that lasted approximately 20 min, with the energy window set to 250–700 KeV. Three-dimensional brain images were subsequently acquired and subjected to Gaussian smoothing. The specific uptake rate (SUR) was calculated using the formula SUR=(Volume of Interest (VOI) of striatum) – (VOI of cerebellum) / VOI of cerebellum [37, 38].
Fast scan cyclic voltammetry (FSCV) for DA dynamics measurements in brain slices
Striatal brain slice preparation. Additional details regarding brain slice preparation can be found in our previous studies [39, 40]. Here, we provide a brief description to allow reproducibility. Following decapitation of the mice, the brain was promptly extracted and immersed in an oxygenated (95% O2/5% CO2) cold cutting solution with the following composition in mM: sucrose 194, NaCl 30, KCl 4.5, MgCl2 1, NaH2PO4 1.2, glucose 10, and NaHCO3 26. After 30 s, the brain tissue was sectioned into 280μm coronal slices, each containing the striatum. Slicing was accomplished using a vibrating blade microtome (VT 100, Leica) within a chamber filled with the cutting solution. Subsequently, brain slices were transferred to a chamber filled with oxygenated artificial cerebrospinal fluid (aCSF; in mM: NaCl 126, KCl 3, MgCl2 1.5, CaCl2 2.4, NaH2PO4 1.2, glucose 11, NaHCO3 26) and incubated at 30°C for 30 min. Following this incubation period, those brain slices were utilized in the FSCV experiments below.
Fast scan cyclic voltammetry (FSCV) for DA measurements in brain slices. The FSCV recording protocols used in this study are also detailed in our previous publications [41, 42]. We employed 280μm coronal brain slices from 22-week-old mice, which were placed in a 0.5 ml chamber at 31–33°C with continuous aCSF perfusion at 2 ml/min. A carbon fiber electrode (7μm diameter; Goodfellow Corp., Oakdale, PA, USA) was precisely inserted between bipolar stimulation electrodes (FHC Inc., Bowdoin, ME, USA) at a depth of 100μm in the dorsal striatum. This carbon fiber electrode assembly was filled with a solution containing 4M potassium acetate and 150 mM KCl. FSCV utilized a triangular waveform (with a scan rate of 400 V/s and a duration of 7 ms), applied every 100 ms, cycling the carbon fiber potential from –0.4 to 1.0 V and then back to –0.4 V. A 5 V voltage stimulus at 25 Hz induced the release of dopamine (DA). Data were collected and analyzed using National Instruments’ A/D board (PCI 6052E and PCI-6711E, National Instruments, Austin, TX, USA) and custom LabView-based software (TarHeel CV, courtesy of Drs. Joseph Cheer and Michael Heien, University of North Carolina, NC, USA). To evaluate the effects of 5-HTP pre-treatment and post-treatment on the DA system in MP LID mice, we measured DA release in the dorsal striatum after pre-infusion of 5-HTP (0.1μM) 20 min followed by L-DOPA and pre-infusion of L-DOPA 20 min followed by 5-HTP. Additionally, we investigated the effect of combined L-DOPA infusion on DA release in the dorsal striatum 20 min after Citalopram (0.1μM) infusion. We compared DA release at 30 min after the administration of various drugs, induced by both 10 pulses (phasic) and a single (tonic) pulse. Electrodes were calibrated with a 0.1μM DA standard solution before and after the experiment to convert current signals into DA concentrations. All signals used in the analysis adhered to the expected voltage-current curve for DA [43].
High performance liquid chromatography (HPLC) analysis of DA and serotonin and their metabolites
The HPLC experimental details are documented in our previous publication [44]. In brief, male mice (22 weeks old) were decapitated 10 min after receiving an injection of L-DOPA (L-DOPA 10 mg/kg and carbidopa 5 mg/kg, i.p.). The dorsal striatum was dissected according to a mouse brain atlas, rapidly frozen using dry ice, and stored at –80°C. Tissues were homogenized in 1 mM oxalic acid and then centrifuged at 15,000 g for 40 min at 4°C. Supernatants were filtered through a 0.22-μm syringe filter (Millipore, Bedford, MA, USA) and injected into the HPLC system for the measurement of DA, 5-HT, and their metabolites [DA metabolites: DOPAC and HVA; serotonin metabolite: 5-HIAA]. Neuronal activity was estimated by calculating DA and 5-HT turnover rates: DA turnover rate=(DOPAC + HVA) / DA, and 5-HT turnover rate = 5-HIAA / 5-HT. Linear regression analysis on a standard curve (10–1,000 nM) was used to determine DOPAC, HVA, DA, 5-HIAA, and 5-HT concentrations by comparing sample measurements with standard values.
The HPLC system was composed of a TSKgel ODS-80TM C18 column (Tosoh, Japan), an LC-10AD pump (Shimadzu, Japan), and an electrochemical detector (Coulochem II, ESA, Chelmsford, MA, USA) equipped with 5010 analytical cells and 5020 guard cells. Analytical cell voltages were set at 40 and 250 mV for detection potential, while the guard cell (mobile phase cell) was maintained at 350 mV. The mobile phase (MDTM mobile phase, ESA) consisted of 75 mM sodium dehydrogenate phosphate (monohydrate), 1.7 mM 1-octanesulfonic acid (sodium salt), 100μl/L triethylamine, 25μM EDTA, and 10% acetonitrile, at a pH of 3.00. It was delivered at a flow rate of 1.0 ml/min.
Western blot analysis
Mice (22 weeks old) were decapitated 10 min post L-DOPA injection (L-DOPA 10 mg/kg and carbidopa 5 mg/kg, i.p.). Subsequently, the dorsal striatum was rapidly dissected according to a mouse brain atlas. Tissues underwent homogenization in RIPA buffer (TAAR-ZBZ5, Biotools Co., Ltd., Taiwan) enriched with a protease inhibitor cocktail (ab20111, Abcam, Cambridge, United Kingdom). Protein concentration was determined using the BCA Protein Assay Kit II (K813, Biovision, Lausen, Switzerland). These proteins were then electrophoresed on a 12% SDS-polyacrylamide gel and electrotransferred onto a polyvinyl difluoride membrane. Following a 45-min incubation in blocking buffer (2% bovine serum albumin and Tris-buffered saline with Tween 20), the membranes were subjected to overnight incubation with primary antibodies: D1 R (1 : 1000, rabbit, GTX100354, GeneTex, Taiwan), pERK (1 : 1000, rabbit, #3179, Cell Signaling Technology, Danvers, MA, USA), ERK (1 : 1000, rabbit, #9102, Cell Signaling Technology, Danvers, MA, USA), pDARPP32 (1 : 1000, rabbit, #5393, Cell Signaling Technology, Danvers, MA, USA), DARPP32 (1 : 1000, rabbit, #2306, Cell Signaling Technology, Danvers, MA, USA), 5-HT1A (1 : 1000, rabbit, GTX02545, GeneTex, Taiwan), 5-HT1B (1 : 1000, rabbit, NB100-56350, Novus, Centennial, CO, USA), and β-actin (1 : 5000, rabbit, ab8227, Abcam, Cambridge, United Kingdom). After three washes, the membranes were exposed to a 1-h incubation with goat HRP-linked anti-rabbit IgG antibodies (1 : 20,000, ab6721, Abcam, Cambridge, United Kingdom). This was followed by development using an ECL detection kit (Amersham Life Sciences, Piscataway, NJ, USA) and imaging with UVP ChemStudio Plus (Analytik Jena AG, Germany). Results were subsequently normalized to the level of β-actin as a loading control, and immunoreactivity intensities were quantified in relation to the corresponding control using NIH Image J.
Statistical analysis
All data were expressed as mean±SEM and subjected to one or two-way analysis of variance (ANOVA), followed by a Bonferroni post hoc test for multiple comparisons, utilizing appropriate software (Prism 6.02; GraphPad, San Diego, CA, USA). A p-value <0.05 was considered statistically significant.
RESULTS
The temporal effect of 5-HTP on the progression of L-DOPA-induced dyskinesia in MP LID mice
Although the serotonergic system is a widely recognized modulator of L-DOPA-induced dyskinesia (LID), the effectiveness of specifically targeting serotonin transporters or receptors in reducing LID in animal models is still uncertain. Hence, in this study, our goal was to further investigate whether time-dependent manipulation of serotonergic neuroplasticity through administration of serotonin precursors or serotonin reuptake inhibitors, based on various temporal regimens, could influence the progression of L-DOPA-induced dyskinesia in L-DOPA-primed MP mice. The modified AIMs [35, 36] were monitored to assess the severity of dyskinesia in L-DOPA-primed MP mice and to determine 5HT drug’s antidyskinetic efficacy (Supplementary Figure 1). In L-DOPA-naive MP mice, a single dose of L-DOPA/carbidopa (MP + L-DOPA) did not result in significant differences in AIM scores when compared to WT mice or MP mice without L-DOPA/carbidopa treatment (Supplementary Figure 2A). However, after a 14-day priming, L-DOPA-treated MP mice exhibited significantly greater LID, as indicated by higher AIM scores after L-DOPA administration (MP LID + Saline + L-DOPA), compared to those without L-DOPA priming (Supplementary Figure 2B, MP + L-DOPA vs. MP LID + Saline + L-DOPA p < 0.001). To explore the role of exogenous serotonin and its interaction with dopamine during the development of LID, 5-HTP, a serotonin precursor, was administered at 30, 60, 90, and 120 min before the injection of L-DOPA/carbidopa in MP LID mice (Fig. 2A). Pre-treatment with 5-HTP 30 min before L-DOPA/carbidopa administration effectively reduced the maximum AIM scores, total AIM scores, as well as AIM scores at 20, 40, and 80 min after L-DOPA/carbidopa treatment (Fig. 2B–D, Baseline vs. 30 min p < 0.05). However, pre-treatment with 5-HTP 60 min before L-DOPA/carbidopa administration did not alter the maximum AIM score in MP LID mice while it only transiently decreased AIM score at 20 min post-L-DOPA/carbidopa. Otherwise, it led to an increase in the total AIM score, along with higher AIM scores at 80, 100, and 120 min after L-DOPA/carbidopa treatment compared to MP LID mice treated with L-DOPA/carbidopa alone (Fig. 2B, 2D, Baseline vs. 60 min p < 0.01). Although pre-treatment with 5-HTP 90 min prior to L-DOPA/carbidopa treatment modestly decreased AIM score 20 min post-L-DOPA/carbidopa, it did not affect maximum or total AIM scores in MP LID mice, similar to the results seen with mice treated with 5-HTP 120 min prior to L-DOPA/carbidopa treatment (Fig. 2B–D). In summary, these results suggest that the timing of serotonin precursor administration plays a crucial role in interfering with abnormal presynaptic dopamine release in L-DOPA-primed mice with significant striatal dopamine denervation. Pre-treatment with 5-HTP 30 min prior to L-DOPA replacement appears to be the most effective therapeutic approach for reducing LID development.
Post-treatment with 5-HTP attenuates established LID
In order to establish the effect of 5-HTP treatment on established LID, MP LID mice received saline or 5-HTP 20 min after L-DOPA/carbidopa treatment (Fig. 3A). Statistical analysis revealed that introduction of 5-HTP significantly reduced total AIM scores, along with lower AIM scores at 80- and 100-min post-L-DOPA/carbidopa administration (Fig. 3B, C, MP LID + L-DOPA + Saline vs. MP LID + L-DOPA + 5-HTP p < 0.001). Considering the previous data on pre-treatment of 5-HTP, these findings imply that 5-HTP treatment has a time-dependent capacity to reduce LID severity in MP LID mice.
Next, we examined whether the administration of 5-HTP could alter locomotor activity induced by L-DOPA/carbidopa administration in MP LID mice. Indeed, MP mice with or without L-DOPA replacement showed significant reductions in both vertical and horizontal activities compared to WT mice, indicating that a single dose of L-DOPA/carbidopa failed to restore hypomobility in MP mice. Following a 14-day L-DOPA/carbidopa priming period, the administration of L-DOPA/carbidopa led to abnormal locomotor hyperactivity (Fig. 3D, E, p < 0.001). Importantly, the administration of 5-HTP 30 min before or 20 min after L-DOPA/carbidopa treatment in MP LID mice could effectively mitigate abnormal rearing movements (Fig. 3D, p < 0.05) with no significant effect on the horizontal hyperactivity (Fig. 3E, p = 0.28). Taken together, 5-HTP treatment not only prevented the development of LID, but also significantly alleviated existing LID in MP LID mice.
5-HTP ameliorates L-DOPA-induced synaptic dopamine release in the striatal slices of MP LID mice
Since the administration of 5-HTP was shown to mitigate LID development and expression, we utilized FSCV to further explore whether the addition of serotonin precursors could temper L-DOPA-derived abnormal DA release in the dorsal striatum. In this experiment, we examined the effects of 5-HTP on the L-DOPA-derived DA release in the dorsal striatum, using WT and MP mice. In the dorsal striatum of intact or compromised dopamine systems, the infusion of L-DOPA alone, but not 5-HTP, resulted in a substantial increase in both tonic and phasic dopamine release (Fig. 4A, 4B; Supplementary Figures 3A, 3B, 4A, 4B, 4E, 4F, 5). While there was no significant difference in baseline DA release between MP and MP LID mice, the infusion of L-DOPA significantly increased DA release in the dorsal striatum of MP LID mice compared to MP mice (Supplementary Figure 3A, B; black rectangle, p < 0.001). In WT mice, pre-treatment with 5-HTP did not alter L-DOPA-induced dopamine release (Supplementary Figure 5). However, in both MP and MP LID mice, the administration of 5-HTP 20 min before L-DOPA infusion notably decreased both tonic and phasic DA release in the dorsal striatum (Supplementary Figure 3C, 3D, 4C, 4D, 4G, 4H, red bar: MP LID + L-DOPA vs. Blue bar: MP LID + 5-HTP + L-DOPA; p < 0.001). In line with pre-treatment findings, the administration of 5-HTP 20 min after L-DOPA infusion markedly diminished the L-DOPA-induced increase in dopamine release in the dorsal striatum of MP or MP LID mice (Supplementary Figure 3E, 3F and 4E-H; red bar: MP LID + L-DOPA vs. green bar: MP LID + L-DOPA + 5-HTP; p < 0.001). In summary, both pre-treatment and post-treatment with 5-HTP demonstrated the ability to alleviate the abnormal increase in both tonic and phasic DA release induced by L-DOPA infusion in the dopamine-denervated dorsal striatum.
Fig. 4
The administration of 5-HTP in the dorsal striatal slices from MP LID mice reduces dopamine release induced by supplementary L-DOPA, as measured using FSCV (fast-scan cyclic voltammetry). Dopamine release following L-DOPA infusion showed a significant increase in MP LID mice in both tonic release (induced by a single pulse at 25 Hz with 5 volts stimulation intensity) and phasic release (induced by 10 pulses at 25 Hz with 5 volts stimulation intensity), as illustrated in graphs (A) and (B) respectively. The increased release of dopamine caused by the infusion of L-DOPA could be suppressed by 5-HTP, whether administered beforehand (C, D) or afterward (E, F). G) With tonic stimulation, the co-administration of 5-HTP effectively reduced L-DOPA-induced dopamine release in the MP LID groups. One-way ANOVA followed by a Bonferroni post hoc test for multiple comparisons. ***p < 0.001, compared to MP LID + L-DOPA (red bar). H) With phasic stimulation, pre-treatment (blue bar, MP LID + 5-HTP + L-DOPA) or post-treatment (green bar, MP LID + L-DOPA + 5-HTP) of 5-HTP exhibited a significant suppression of L-DOPA-induced dopamine release in striatal slices. One-way ANOVA followed by a Bonferroni post hoc test for multiple comparisons. ***p < 0.001, compared to MP LID + L-DOPA; MP LID + 5-HTP + L-DOPA compared to MP LID + L-DOPA + 5-HTP, #p < 0.05.
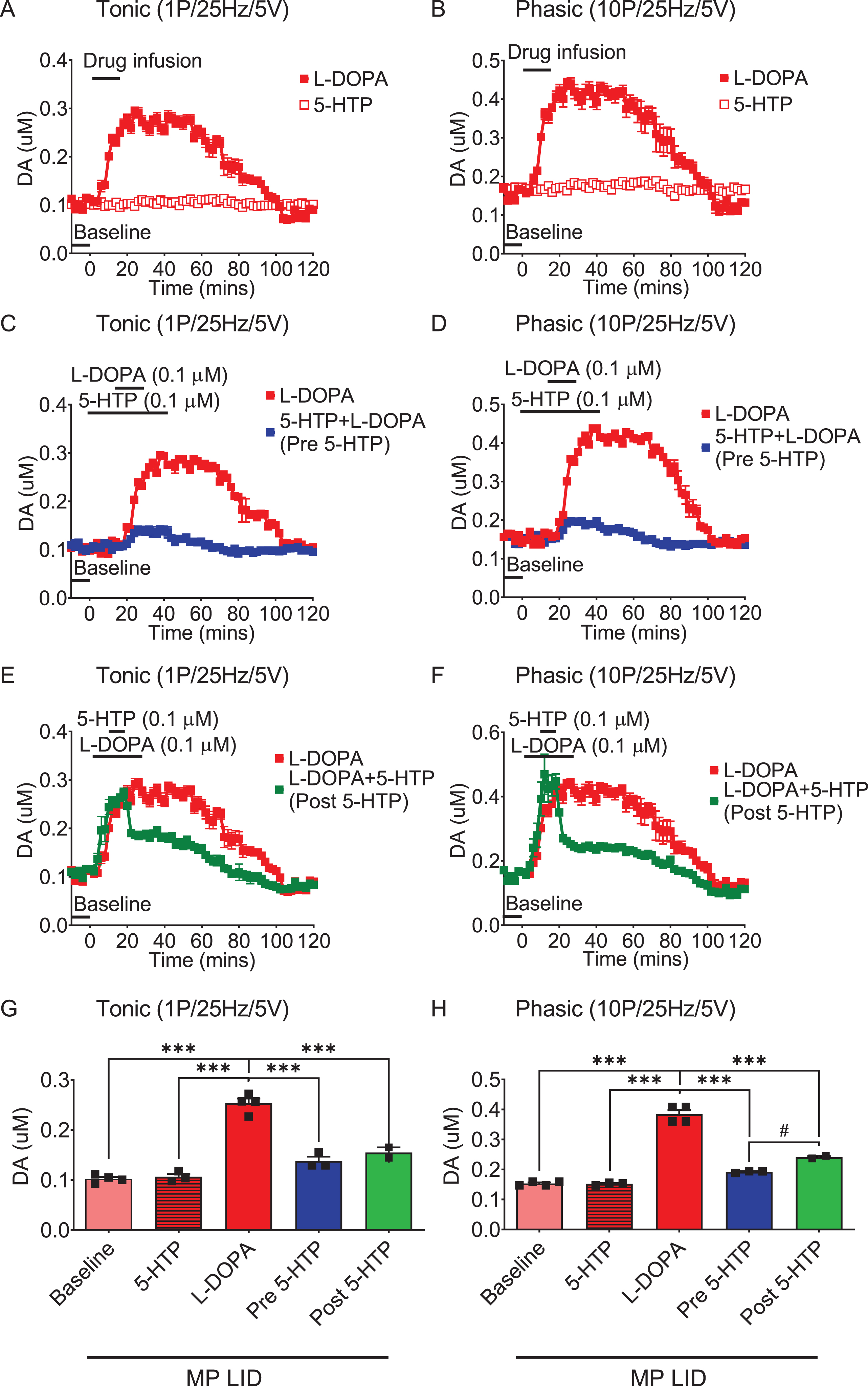
Comparative assessment of the effect of a 5-HT precursor and a SERT inhibitor on established LID in L-DOPA-primed MP mice
Although SERT inhibitor treatment, which elevates 5-HT levels similar to the administration of 5-HT precursors, has been demonstrated to mitigate LID in animal models of PD, there is limited understanding of the disparity between the effects of SERT inhibitors and 5-HT precursors on L-DOPA-induced AIMs. In this study, we further investigated whether the SERT inhibitor Citalopram possesses specific effects on LID in MP LID mice (Fig. 5A). Consistent with the effects of 5-HTP administration, which lowered the total AIM scores in MP LID mice following L-DOPA/carbidopa administration, pre-treatment with Citalopram also significantly reduced the total AIM scores (Fig. 5B, C, MP LID + Saline + L-DOPA vs. MP LID + Citalopram + L-DOPA p < 0.01). Notably, pre-treatment with Citalopram particularly improved (i.e., lowered) abnormal axial scores of MP LID mice (Fig. 5D; MP LID + Saline + L-DOPA vs. MP LID + Citalopram + L-DOPA p < 0.05), whereas pre-treatment with 5-HTP predominantly alleviated the limb as well as standing scores (Fig. 5E, F; MP LID + Saline + L-DOPA vs. MP LID + 5-HTP + L-DOPA p < 0.05). In the rearing test, both pre-treatment with Citalopram and pre-treatment with 5-HTP effectively improved (i.e., reduced) the abnormal vertical activity of MP LID mice after L-DOPA/carbidopa administration (Fig. 5G, p < 0.01). Taken together, treatment with either a SERT inhibitor or an 5-HT precursor attenuated established L-DOPA-induced AIMs and vertical hyperactivity. However, the SERT inhibitor predominantly mitigated abnormal body axial movements, while the 5-HT precursor alleviated limb AIMs in L-DOPA-induced dyskinesia.
Fig. 5
Pharmacological modulation of the serotonin system can potentially affect the release of dopamine that is implicated in the development and manifestation of LID in MP mice. In addition to 5-HTP, Citalopram, a selective serotonin reuptake inhibitor (SERT inhibitor), was co-administered with L-DOPA. Subsequently, the AIM score was monitored using the experimental protocol depicted in (A). B) The AIM scores of each group were documented every 20 min following the injection of L-DOPA, over a duration of 2 h. C) The administration of 5-HTP (red bar: MP LID + Saline + L-DOPA vs. blue bar: MP LID + 5-HTP + L-DOPA, p < 0.01) or Citalopram (red bar: MP LID + Saline + L-DOPA vs. purple bar: MP LID + Citalopram + L-DOPA, p < 0.01) resulted in a significant decrease in the total AIM scores in L-DOPA-primed MP. D) The administration of Citalopram (p < 0.05) suppresses the occurrence of abnormal axial movements in the MP LID mice. The abnormal increase in (E) limb movements and (F) standing behavior observed in MP LID mice can be mitigated by 5-HTP (p < 0.05). G) The administration of either 5-HTP or Citalopram ameliorates the abnormal increase in vertical activity in MP LID mice. One-way ANOVA followed by a Bonferroni post hoc test for multiple comparisons. *p < 0.05, **p < 0.01, ***p < 0.001, compared to MP LID + Saline + L-DOPA.
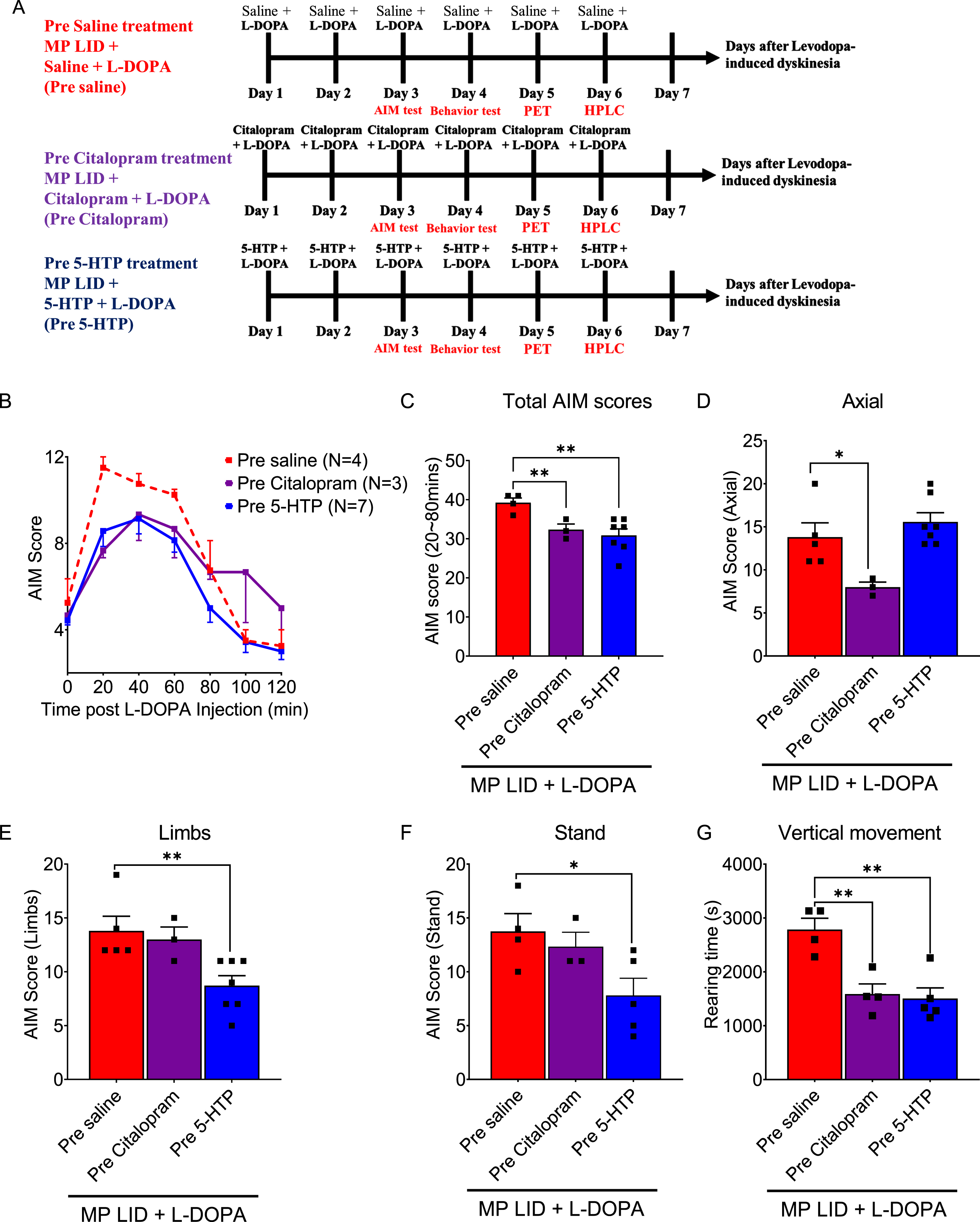
Infusion of Citalopram decreases the synaptic dopamine release induced by L-DOPA administration in the striatal slices of MP LID mice
Considering that SERT inhibitors and the 5-HT precursor led to declines in distinct AIM subtypes in MP LID mice, our next objective was to explore whether the administration of Citalopram induces alterations in dopamine release from the synaptic cleft in the dorsal striatum of MP LID mice (Fig. 6). The infusion of Citalopram alone had no effect on dopamine release in the intact or compromised dorsal striatum (Supplementary Figures 4C–F). However, in both MP and MP LID mice, the addition of Citalopram 20 min before L-DOPA infusion attenuated the L-DOPA-induced increase in dopamine release in striatal slices (Supplementary Figures 3G, 3H, 5, and Fig. 6A–D; MP + L-DOPA vs. MP + Citalopram + L-DOPA p < 0.05; red bar: MP LID + L-DOPA vs. purple bar: MP LID + Citalopram + L-DOPA p < 0.05). Accordingly, the inhibitory effect of Citalopram on L-DOPA-induced increase in DA release in the DA-denervated striatum is comparable to that of 5-HTP treatment (Fig. 6C, D). The comparison of Pre-Citalopram with Pre-5-HTP showed p-values of 0.4848 in the tonic phase and 0.0930 in the phasic phase.
Fig. 6
DA release in striatal slices detected by FSCV. Both L-DOPA-induced increments in tonic (A) and phasic (B) release were reduced after Citalopram (0.1μM) infusion in brain slices from MP LID groups. Overall, the administration of 5-HTP or Citalopram, effectively suppressed both tonic (C) and phasic (D) dopamine release induced by L-DOPA infusion in the MP LID group. One-way ANOVA followed by a Bonferroni post hoc test for multiple comparisons. *p < 0.05, **p < 0.01, ***p < 0.001, compared to MP LID + L-DOPA.
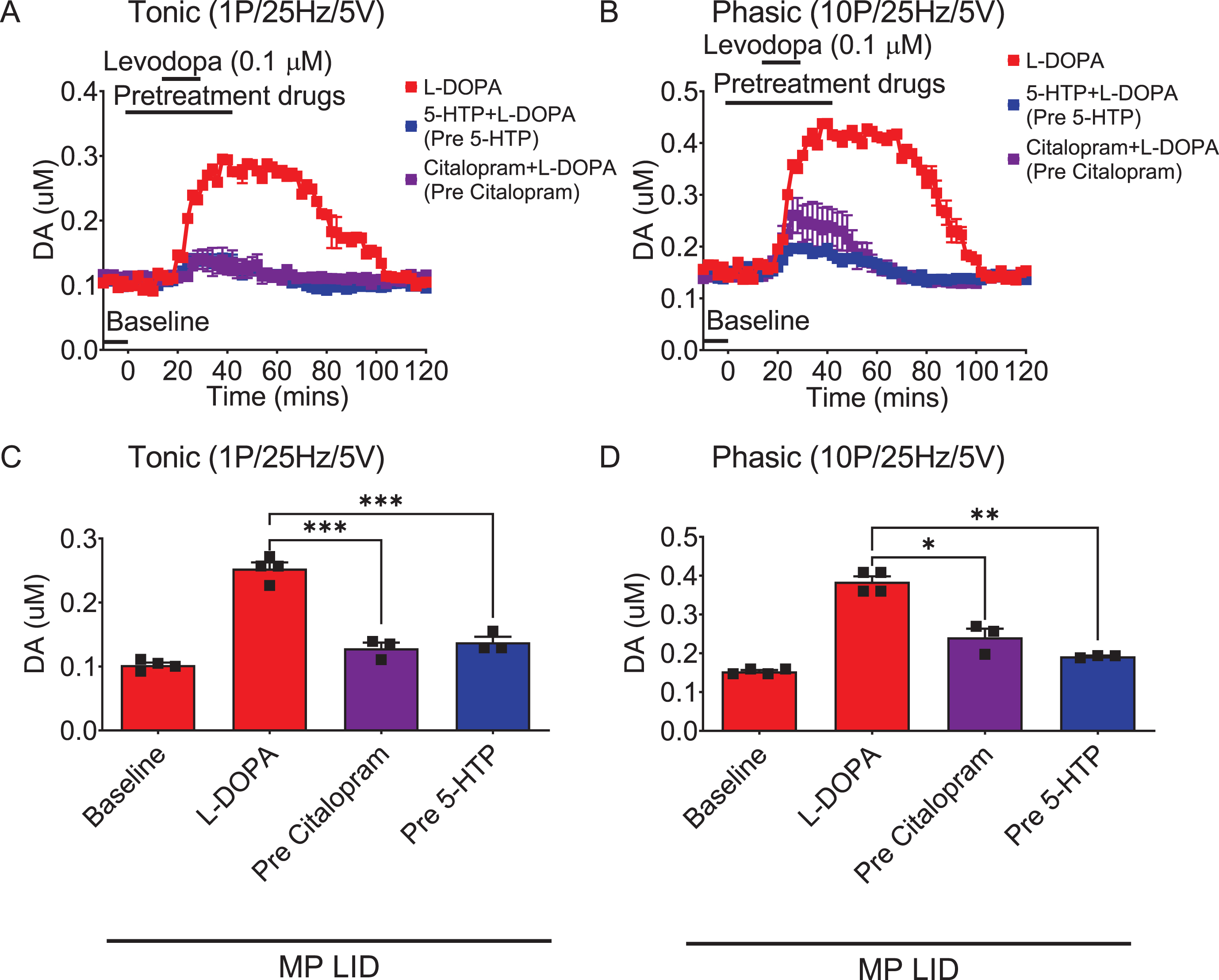
5-HTP treatment, but not Citalopram, modulates abnormal dopamine turnover in the dorsal striatum of MP LID mice
As 5-HTP and Citalopram exhibited similar effects on DA release in striatal slices during L-DOPA infusion, we next aimed to determine whether the differences in AIM subtypes observed in MP LID mice following these two drugs were associated with concentrations of dopamine, serotonin, and their metabolites in the dorsal striatum (Fig. 7). The concentrations of dopamine and its metabolites in the dorsal striatum of 22-week-old MP mice were significantly lower (Fig. 7A–C), but its DA turnover rate was significantly higher (Fig. 7D) compared to WT mice of the same age. Although MP LID mice exhibited a significant increase in the concentration of DA and its metabolites compared to MP mice without L-DOPA replacement (Fig. 7A–C), there was, however, no change in the DA turnover rate between the two groups (Fig. 7D). Before elucidating the roles of 5-HTP or Citalopram on the concentration of dopamine and its metabolites in the MP LID mice, our aim was to examine the individual effects of 5-HTP or Citalopram in WT or MP mice. The administration of 5-HTP alone did not affect the concentrations of DA and its metabolites in the dorsal striatum of WT or MP mice. However, 5-HTP as well as Citalopram significantly increased serotonin and its metabolite concentrations (Supplementary Figure 7). Notably, treatment with Citalopram alone was shown to increase the concentrations of DOPAC and HVA in WT mice, suggesting that SERT inhibition by Citalopram may accelerate DA metabolism in healthy dopaminergic neurons. Pre-treatment with 5-HTP in MP LID mice resulted in a significant reduction in the concentration of L-DOPA-derived DA and its metabolites as shown in Fig. 7A–C, (p < 0.01). This led to a decreased DA turnover rate in the dorsal striatum compared to MP LID mice (Fig. 7D, p < 0.01). In contrast, it increased the concentrations of 5-HT and its turnover rate in the dorsal striatum of MP LID mice. In contrast to the results noted with 5-HTP treatment, a single injection of Citalopram as a pre-treatment in MP LID mice did not reduce L-DOPA-upregulated DA turnover rate in the dorsal striatum. However, it did result in a significant reduction in the concentrations of DA and HVA as shown in Fig. 7A and 7C, (p < 0.01). This suggests that SERT inhibition by Citalopram may reduce the release of L-DOPA-derived DA in the DA-denervated striatum, leading to the conversion of DA to generate DOPAC in pre-synaptic neurons. Surprisingly, Citalopram treatment did not increase the concentrations of 5-HT and its metabolites in the dorsal striatum of MP LID mice (Fig. 7E–G). This suggests that chronic replacement of L-DOPA in MP mice may promote SERT availability, potentially interfering with the blockade by Citalopram of 5-HT reuptake.
Fig. 7
The concentrations of DA, serotonin, and their metabolites in the striatum were measured using high-performance liquid chromatography (HPLC) in the presence or absence of L-DOPA treatment. A) The DA concentration in the striatum of MP mice was lower than that of WT mice. The DA concentration in the striatum of MP LID mice receiving L-DOPA was higher than that in MP mice. The significant increase in DA concentration induced by L-DOPA injection in the MP LID group can be effectively suppressed by pre-treatment with 5-HTP or Citalopram. Only the administration of 5-HTP was able to suppress the increase in dopamine metabolites (red bar: MP LID + Saline + L-DOPA vs. blue bar: MP LID + 5-HTP + L-DOPA, p < 0.01), including DOPAC (p < 0.001) (B) and HVA (p < 0.001) (C), in the MP LID group following L-DOPA administration. The administration of Citalopram reduces only the increase in tissue concentration of HVA induced by L-DOPA in the MP LID group. D) The DA turnover rate increased significantly in MP and MP LID groups, which was suppressed by the administration of 5-HTP (p < 0.01). The concentration of serotonin (p < 0.05) (E), 5-HIAA (p < 0.01) (F), and the 5-HT turnover rate (p < 0.01) (G) exhibited significant increases in the striatum in MP LID mice given 5-HTP and L-DOPA, compared to MP LID mice given saline and L-DOPA. However, the administration of Citalopram did not have any effect on these measures. One-way ANOVA followed by a Bonferroni post hoc test for multiple comparisons. *p < 0.05, **p < 0.01, ***p < 0.001, compared to MP LID + Saline + L-DOPA; MP compared to MP LID + Saline + L-DOPA, #p < 0.05, ###p < 0.001; WT compared to MP, $$$p < 0.001.
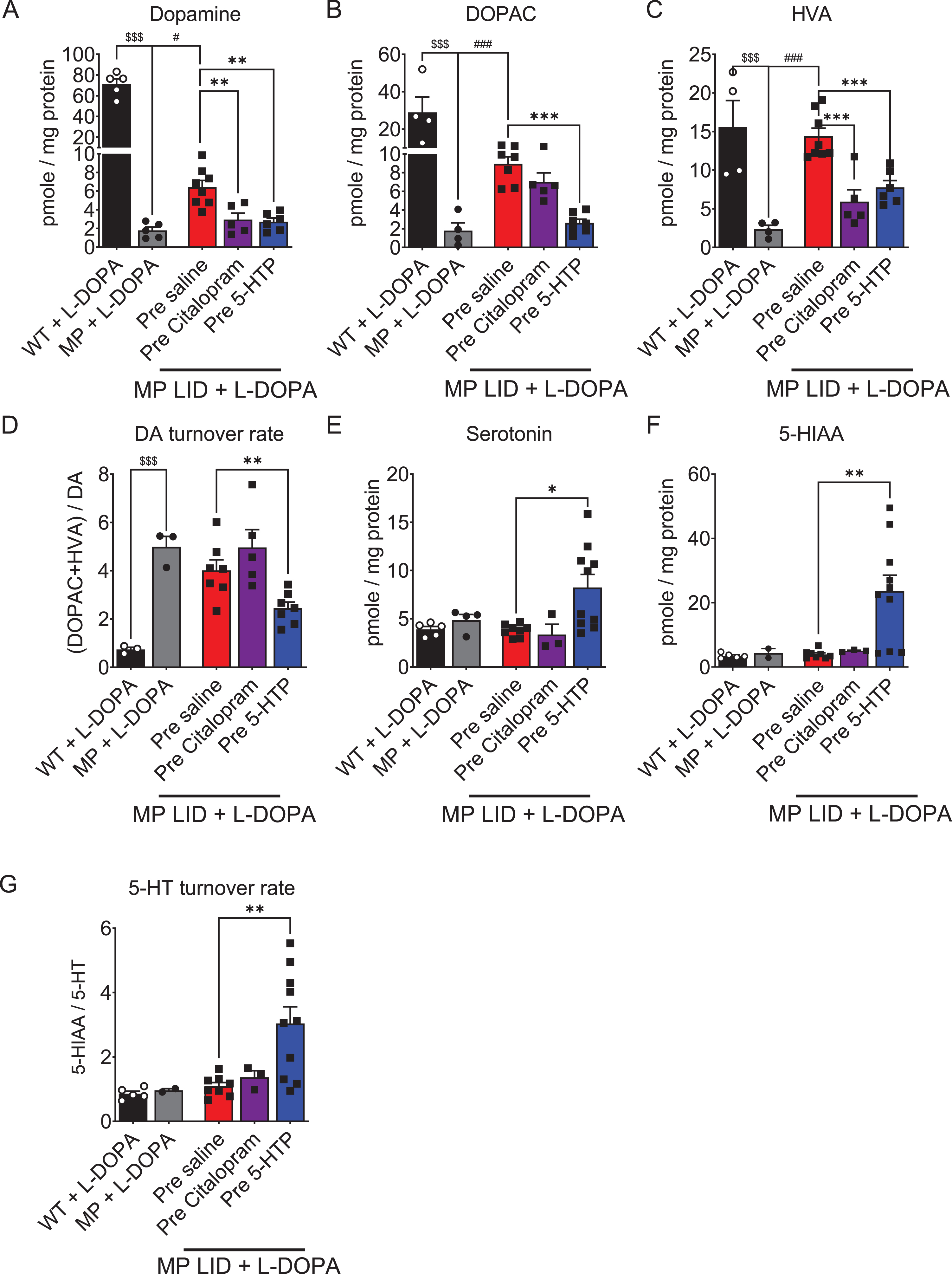
Next, we investigated the relationship between AIM scores and neurotransmitter levels in the dorsal striatum using linear regression (Supplementary Figure 8). Statistical analysis revealed a strong correlation between dorsal striatal DA concentration and AIM total scores, with an R2 value of 0.8323 (Supplementary Figure 8A). In contrast, AIM total scores exhibited relatively weaker correlations with DA turnover rate (Supplementary Figure 8B, R2 = 0.006302), serotonin concentration (Supplementary Figure 8C, R2 = 0.0000006317), 5-HT turnover rate (Supplementary Figure 8D, R2 = 0.02332), and DA turnover rate/5-HT turnover rate (Supplementary Figure 8E, R2 = 0.0000001995). This suggests that the total AIM score may be correlated with DA concentrations in the dorsal striatum rather than 5-HT concentrations. However, the concentrations of DOPAC and the turnover rates of 5-HT displayed robust correlations with abnormal limb movement (Supplementary Figure 8E, R2 =0.998) and standing movement (Supplementary Figure 8F, R2 = 0.9948), indicating that variations in dopamine metabolites and serotonin turnover rates in L-DOPA-primed MP mice might play a role in distinct subtypes of LID after treatment with these two serotoninergic drugs.
5-HTP treatment attenuates hyperactivation of the D1 R signaling pathway in the dorsal striatum of MP LID mice
In L-DOPA-primed MP mice, a notable elevation in the expression of phosphorylated ERK (p-ERK) or phosphorylated DARPP-32 (p-DARPP-32) was detected in the dorsal striatum. Additionally, there was a trend towards increased expression of D1 receptor protein (Fig. 8A–D). The observed upregulation of the D1 receptor signaling pathway could potentially be linked to the development of L-DOPA-induced dyskinesia [45]. A 30-min pre-treatment of 5-HTP followed by L-DOPA/carbidopa administration in MP LID mice downregulated the L-DOPA-increased expressions of p-ERK and p-DARPP-32 (Fig. 8C, D, p < 0.01). In contrast, pre-treatment with Citalopram followed by L-DOPA/carbidopa administration exhibited little effect on the L-DOPA-induced upregulation of the D1 signaling pathway in MP LID mice (Fig. 8C, D). Single injection of 5-HTP or Citalopram did not affect the expression of either the 5-HT1A or 5-HT1B receptors in the dorsal striatum of MP LID mice (Fig. 8E, F, p = 0.47). Taken together, the treatment with 5-HTP, which directly increases 5-HT levels, significantly mitigates the L-DOPA-induced upregulation of the D1 downstream signaling pathway in the DA-denervated striatum.
Fig. 8
The expression of proteins involved in dopaminergic and serotonergic transmission was analyzed in the striatum of WT, MP, and MP LID mice following co-treatment of L-DOPA with 5-HTP or Citalopram. A) Dopaminergic and serotonergic transmission-related protein expression in the striatum were illustrated. B) There was no significant change in D1 receptor protein expression among these groups. However, the administration of 5-HTP effectively suppressed the increased phosphorylation of signaling molecules in the downstream D1 pathway, specifically ERK (red bar: MP LID + Saline + L-DOPA vs. blue bar: MP LID + 5-HTP + L-DOPA, p < 0.01) (C) and DARPP32 (p < 0.01) (D), in the MP LID mice. In contrast, the administration of Citalopram did not have a significant effect on the phosphorylation levels of these molecules. There were no significant changes in the expressions of 5-HT1A receptor (E) and 5-HT1B receptor (F) proteins among these groups. One-way ANOVA followed by a Bonferroni post hoc test for multiple comparisons. **p < 0.01, compared to MP LID + Saline + L-DOPA; MP compared to MP LID + Saline + L-DOPA, ##p < 0.01.
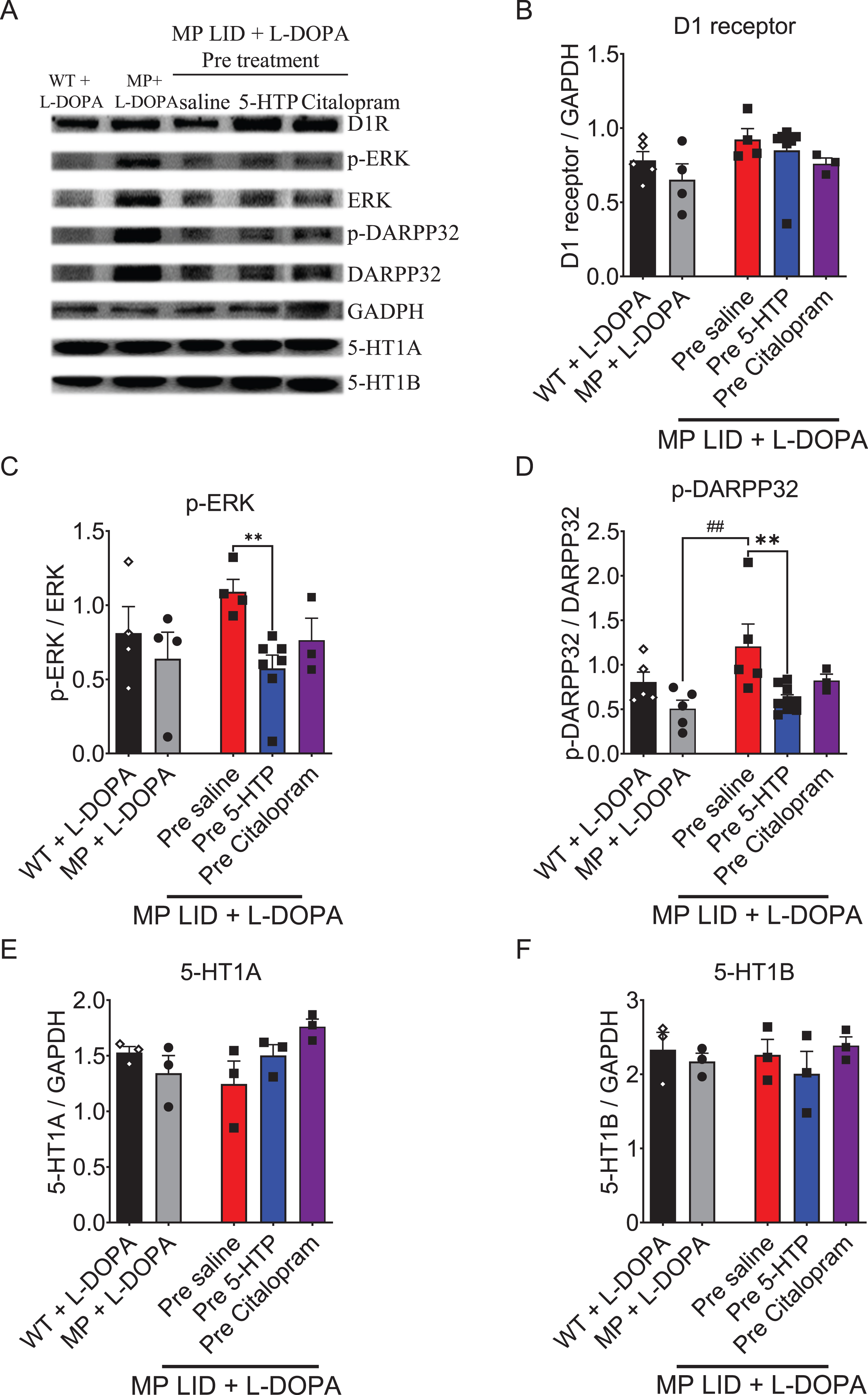
Citalopram treatment downregulates SERT availability in the dorsal striatum of L-DOPA-primed MP mice
Since previous studies suggested abnormal SERT function is implicated in LID development [46, 47], we next investigated SERT activity in MP LID mice treated with or without 5-HT-targeting drugs followed by L-DOPA/carbidopa replacement (Fig. 9A). This evaluation was conducted using [18F]FE-ADAM radioactivity (SUR) measurements obtained through PET scans. SERT availability in the dorsal striatum of MP mice at 22 weeks was significantly lower than that in WT mice (Fig. 9B, p < 0.01). Following a 14-day treatment with L-DOPA/carbidopa, there was a significant upregulation in SERT availability in MP mice, particularly in those exhibiting higher AIM scores (as depicted in Fig. 9B, p < 0.001). Pre-treatment with 5-HTP followed by administration of L-DOPA/carbidopa in MP LID mice did not result in any significant alteration in SERT availability in the dorsal striatum, compared to the saline treated group. In contrast, pre-treatment with Citalopram effectively reduced the increased SERT availability induced by L-DOPA in the dorsal striatum of MP LID mice (Fig. 9B, p < 0.05). Collectively, these findings further support the concept that DA denervation allows serotonergic neurons to play major roles in the conversion of L-DOPA into DA and reuptake of DA through SERT [48, 49]. Prolonged L-DOPA replacement further enhances serotonergic neuroplasticity, reinforcing SERT availability and contributing to LID development. Importantly, this aberrant cycling of exogenous L-DOPA-derived extracellular DA appears to be reversible with a single injection of a SERT inhibitor.
Fig. 9
PET scans of serotonin transporter (SERT) activity in WT, MP and MP LID mice. A) The bioactivity or availability of the serotonin transporter (SERT) was assessed using [18F]FE-ADAM radioactivity (SUR) measured in PET scans. Experimental animals were given L-DOPA and carbidopa for 20 min before undergoing PET scans. A lower activity was seen in MP mice compared to WT mice (WT + L-DOPA vs. MP + L-DOPA, p < 0.01), while an increase in activity was observed in MP LID mice. B) The SUR values in each group, plotted on a panel, revealed that the availability of SERT in MP LID mice was higher compared to MP mice (MP + L-DOPA vs. MP LID + Saline + L-DOPA, p < 0.001). Citalopram treatment significantly attenuated the increased SERT availability in MP LID mice (MP LID + Saline + L-DOPA vs. MP LID + Citalopram + L-DOPA, p < 0.05). However, the administration of 5-HTP did not result in any significant effect on the availability of SERT in MP LID animals. One-way ANOVA followed by a Bonferroni post hoc test for multiple comparisons. MP LID + Saline + L-DOPA compared to MP LID + Citalopram + L-DOPA, *p < 0.05; MP + L-DOPA compared to MP LID + Saline + L-DOPA, ###p < 0.001; WT + L-DOPA compared to MP + L-DOPA, $$p < 0.01.
![PET scans of serotonin transporter (SERT) activity in WT, MP and MP LID mice. A) The bioactivity or availability of the serotonin transporter (SERT) was assessed using [18F]FE-ADAM radioactivity (SUR) measured in PET scans. Experimental animals were given L-DOPA and carbidopa for 20 min before undergoing PET scans. A lower activity was seen in MP mice compared to WT mice (WT + L-DOPA vs. MP + L-DOPA, p < 0.01), while an increase in activity was observed in MP LID mice. B) The SUR values in each group, plotted on a panel, revealed that the availability of SERT in MP LID mice was higher compared to MP mice (MP + L-DOPA vs. MP LID + Saline + L-DOPA, p < 0.001). Citalopram treatment significantly attenuated the increased SERT availability in MP LID mice (MP LID + Saline + L-DOPA vs. MP LID + Citalopram + L-DOPA, p < 0.05). However, the administration of 5-HTP did not result in any significant effect on the availability of SERT in MP LID animals. One-way ANOVA followed by a Bonferroni post hoc test for multiple comparisons. MP LID + Saline + L-DOPA compared to MP LID + Citalopram + L-DOPA, *p < 0.05; MP + L-DOPA compared to MP LID + Saline + L-DOPA, ###p < 0.001; WT + L-DOPA compared to MP + L-DOPA, $$p < 0.01.](https://ip.ios.semcs.net:443/media/jpd/2024/14-5/jpd-14-5-jpd240080/jpd-14-jpd240080-g009.jpg)
DISCUSSION
In this study, we first evaluated the therapeutic potential of 5-HTP in the developing or established LID in a mouse model of progressive PD. In addition, we analyzed the dynamics of DA release in the DA-denervated striatum following the addition of L-DOPA and serotonergic drugs. Furthermore, we compared the effects of 5-HTP and a SERT inhibitor, Citalopram, to clarify the correlation between abnormal involuntary movements and the levels of DA, 5-HT, and their respective metabolites.
In previous literature, the underlying mechanism of LID has been suggested as an abnormal increase in DA in the dorsal striatum following chronic replacement of L-DOPA [26, 50]. Furthermore, it has been suggested that aromatic L-amino acid decarboxylase (AADC) and vesicular monoamine transporter 2 (VMAT2) within the serotonin system may have a significant influence on DA production in the context of nigrostriatal dopaminergic neuron loss [51]. Together these findings suggest that the 5-HT neurons can serve as alternatives to the damaged DA pathway, converting L-DOPA into DA for release in the striatum. However, this serotonergic neuroplasticity can be maladaptive in the late stage of PD with chronic replacement of L-DOPA [47]. Aberrant transmitter release of DA from serotonin neurons is considered to be a key initiator of LID, working in concert with the intermittent supplementation of L-DOPA to generate pulsatile stimulation of striatal DA receptors [12, 47, 52–54] (Fig. 10A). Thus, medications acting on SERT or 5-HT1A receptors demonstrate a significant reduction in LID development and expression [11]. However, the effects of serotonergic agents on the dynamic levels of DA, 5-HT, and their metabolism in the DA-denervated striatum remained unclear. In this study, MP mice were used to recapitulate several features of PD in humans, such as adult-onset degeneration of nigrostriatal dopamine circuitry and motor deficits that are ameliorated by L-DOPA administration. Based on our previous data [55, 56], all DA neurons that express DAT and TH experienced significant loss, including both those projecting to striatal and those projecting to extrastriatal regions, in MP mice aged up to 20 weeks. Accordingly, MP mice, modeling both behavioral and biochemical characteristics of long-term L-DOPA treatment in PD patients provide a novel, consistent and progressive animal model of dyskinesia to investigate the role of the serotonin system in LID development.
Fig. 10
Graphical illustration delineates the effects of 5-HTP and Citalopram on DA release induced by the administration of L-DOPA in MP LID mice. A) The administration of L-DOPA induces aberrant release of DA in the synaptic cleft. The release of L-DOPA-derived DA may be regulated by the 5-HT neurons. B) Through the administration of 5-HTP, which competes with L-DOPA for AADC, the amount of DA in synaptic vesicles is reduced, thereby partially normalizing the abnormal DA levels in the synaptic cleft. Moreover, 5-HTP-derived 5-HT could activate 5-HT1 receptors, subsequently downregulating serotonin neuron-derived DA release in the DA-denervated striatum. C) Citalopram, by inhibiting SERT activity, may impede the reuptake of DA into presynaptic serotonin neurons, which attenuates the L-DOPA-induced aberrant DA release in the DA-denervated striatum. In addition, Citalopram administration increases serotonin concentration in the synaptic cleft by inhibiting SERT reuptake. Subsequently, excess 5-HT activates 5-HT1 receptors, subsequently inhibiting 5-HT neuron activity and reducing serotonin neuron-derived DA release after L-DOPA administration.
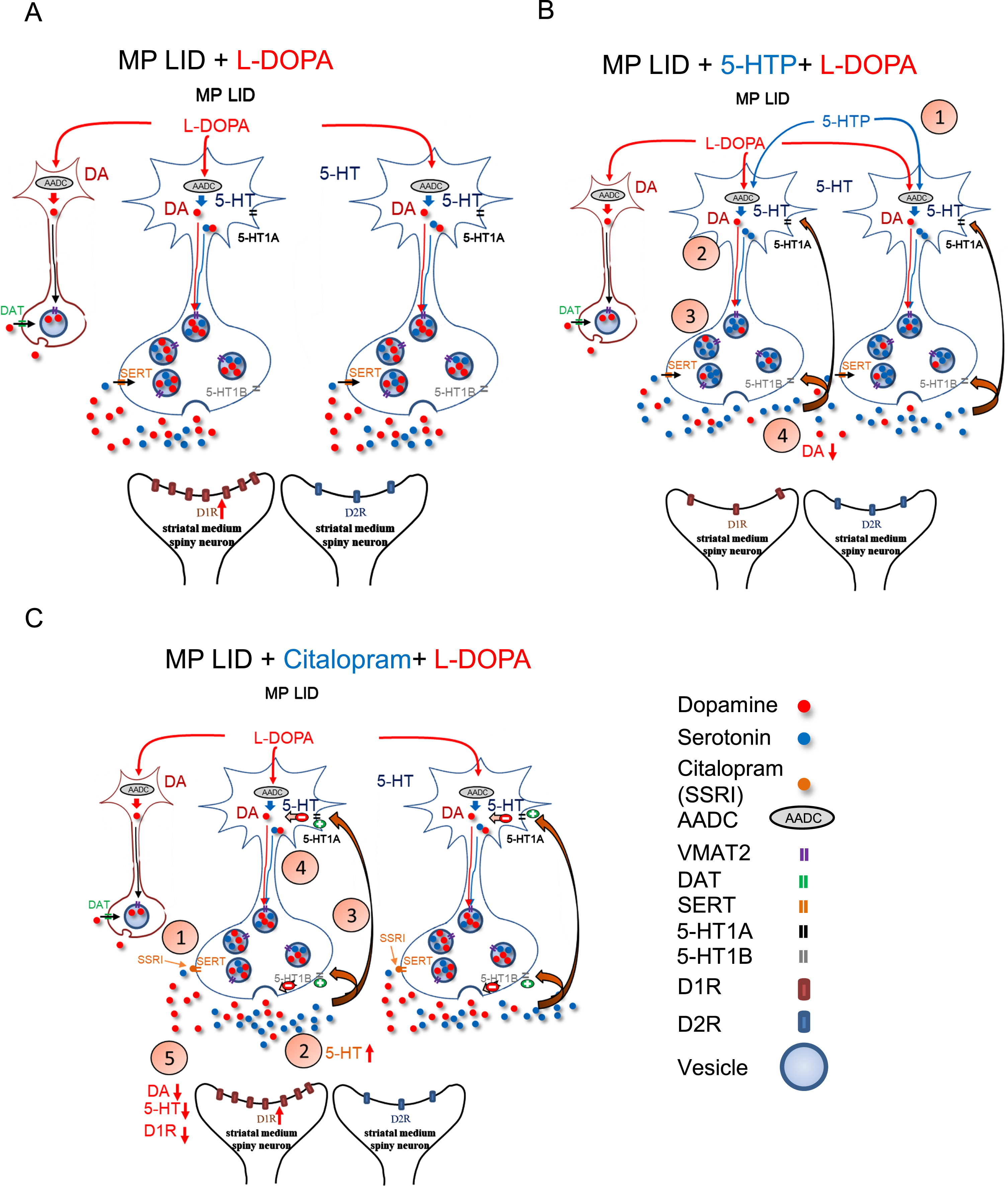
5-HTP is becoming a significant drug in LID research due to its ability to elevate 5-HT levels, thereby preserving 5-HT-mediated signaling and selectively modifying biochemical cascades associated with dyskinetic movements [24, 57]. Previous studies have shown that the anti-dyskinetic effects of 5-HTP are mediated by both a partial displacement of L-DOPA-derived DA storage into serotonergic vesicles, and 5-HT1 receptor activation [24]. Although false transmitter release of 5-HT from presynaptic terminals after administration of 5-HTP seems not to affect the effectiveness of L-DOPA [25], the prolonged activation of 5-HT1 receptors could potentially diminish L-DOPA’s capacity to maintain motor activity in advanced stages of PD [58]. Hence, in this study, we utilized a single injection of 5-HTP to assess the immediate impact of 5-HTP on the development of LID. Interestingly, a single administration of 5-HTP exhibited time-dependent anti-dyskinetic properties and attenuated the surge release of L-DOPA-derived DA in the DA-denervated striatum. Considering that 5-HTP was shown to easily cross the blood-brain barrier without competition from L-DOPA [23], our results further highlight that the application of 5-HTP has the capacity to dampen aberrant serotonin neuron-derived DA release in the DA-denervated striatum (Fig. 10B) [24]. Importantly, treatment with 5-HTP after the onset of LID was also able to decrease established AIMs without compromising the improvements induced by L-DOPA in horizontal movements (Supplementary Figure 6). This implies that a single injection of 5-HTP could potentially serve as a treatment strategy to quickly attenuate the sudden onset of LID in PD patients receiving L-DOPA treatment.
While previous study showed the alleviation of LID by 5-HTP occurring through the downregulation of hyperactivated striatal AKT/mTOR/S6K and CREB/ΔFosB signaling [59], our findings indicated that an injection of 5-HTP significantly diminishes the elevated activation of D1/DARPP32/ERK in the striatum of LID MP mice. This small difference might be attributed to the possibility that long-term administration of 5-HTP could affect downstream pathways, like mTOR signaling associated with synaptic plasticity [59]. However, the immediate effect of 5-HTP on the DA-denervated striatum depends on optimizing the quantal release of 5-HT and L-DOPA-derived DA, either in the serotonergic or remaining dopaminergic neurons within the striatum. This acute effect in mitigating the surge release of L-DOPA-derived DA by 5-HTP treatment may contribute directly to the hyperactivation in downstream pathways of the D1 receptor.
In contrast to the pharmacological mechanism of 5-HTP, a SERT inhibitor, Citalopram, functions by blocking 5-HT reuptake to elevate synaptic 5-HT; this indirectly activates 5-HT1 somatodendritic autoreceptors, thereby inhibiting L-DOPA-derived DA release in serotonin neurons within the DA-denervated striatum (Fig. 10C) [17, 60–65]. In this study, we showed that a single injection of Citalopram reduces total AIM scores in LID MP mice, similar to the effects observed with 5-HTP treatment. In an analysis of each subtype of AIMs, we observed that Citalopram alleviates abnormal axial twisting but does not diminish the involuntary limb and standing movements induced by L-DOPA treatment. Additionally, Citalopram does not affect the activation of D1/DARPP32/ERK in the striatum of LID MP mice, while it exhibits a negative feedback mechanism on L-DOPA-derived dopamine release as indicated by FSCV analysis. The differences in the improvement of subtypes of L-DOPA-induced abnormal movements leads to hypotheses on whether the two serotonergic drugs induce variations in the levels or metabolism of DA and 5-HT in the DA-denervated striatum. In WT mice, both 5-HTP and Citalopram demonstrated the ability to elevate 5-HT levels without affecting DA levels in the striatum (Supplementary Figure 7A). However, Citalopram, through the activation of 5-HT1 autoreceptors, interferes with dopaminergic regulation of the raphe-striatal pathway, leading to an increase in the metabolism of DA to DOPAC and HVA (Supplementary Figure 7B, C). In L-DOPA-naive or L-DOPA-primed MP mice, there was an observed increase in the DA turnover rate in the DA-denervated striatum, aligning with findings from previous studies. While these two serotonergic drugs could decrease DA levels in the striatum of LID MP mice, only 5-HTP exhibited the capacity to further decrease DOPAC and HVA levels, thereby downregulating DA turnover rate in the striatum. 5-HTP also demonstrated the ability to upregulate 5-HT turnover rates in the striatum of LID MP mice. In our linear regression analysis, the severity of standing and vertical movement induced by L-DOPA injection strongly correlated with DOPAC concentration and the ratio of DA to 5-HT, respectively (Supplementary Table 2; Supplementary Figure 8E). However, the level of limb involuntary movements showed a reverse correlation with 5-HT turnover rate (Supplementary Table 2; Supplementary Figure 8F), suggesting that 5-HT metabolism might play a role in the development or expression of LID. Those correlations suggest that 5-HTP, as opposed to Citalopram, reduces DOPAC concentration and upregulates 5-HT turnover rate, thereby mitigating the severity of involuntary standing and limb movements induced by L-DOPA treatment, respectively. Thus, 5-HTP, through simultaneously regulating DA and 5-HT metabolism, exhibits more promising anti-dyskinetic efficacy.
However, in this study, we did not address the potential occurrence of serotonin syndrome resulting from an elevated level of 5-HT due to the chronic administration of 5-HTP treatment. Additionally, we did not investigate the effects of serotonergic drugs on non-motor symptoms in this L-DOPA-primed animal model. Furthermore, whether the ratio of DA to 5-HT in the striatum, which is altered in PD, interferes with the effects of serotonergic agents on LID remains an open question.
Nonetheless, we show here that single injection of 5-HTP or a SERT inhibitor disrupts the development or establishment of L-DOPA-induced AIMs. The immediate anti-dyskinetic effects of 5-HTP and Citalopram are achieved by fine-tuning the quantal release of L-DOPA-derived DA in the DA-denervated striatum. Importantly, single administration of 5-HTP can particularly attenuate the L-DOPA-induced abnormal limb and standing movements without compromising L-DOPA’s improvement in the horizontal movement throughout the treatment period. This may be mediated by decreasing striatal DA turnover rate but increasing 5-HT metabolism after L-DOPA administration. While several questions remain regarding the interplay of monoamine metabolism and dyskinetic development, this study establishes a novel role for serotonergic drugs for improving the use of L-DOPA therapy in PD.
ACKNOWLEDGMENTS
The authors have no acknowledgments to report.
FUNDING
This research is supported by The Ministry of Science and Technology of Taiwan, ROC, MOST 111-2314-B-016 -051 -MY3, MOST 111-2314-B-016-057 and NSTC 112-2314-B-016 -068 -MY3; Medical Research Project grants TSGH-D-112095, and TSGH-C01-112024 from the Tri-Service General Hospital of Taiwan; Medical Research Project grants TSGH-SS_E_113010 from the Tri-Service General Hospital Songshan Branch of Taiwan; The National Defense Medical Center, ROC, MAB_D_112004, MND-MAB-C05-112019, and grant MND-MAB-C10-113034; The Intramural Research Program of the National Institute on Aging, National Institutes of Health, USA. The Swedish Research Council, the Swedish Brain Foundation.
CONFLICT OF INTEREST
The authors have no conflict of interest to report.
DATA AVAILABILITY
Data sharing is not applicable to this article as no datasets were generated or analyzed during this study.
SUPPLEMENTARY MATERIAL
[1] The supplementary material is available in the electronic version of this article: https://dx.doi.org/10.3233/JPD-240080.
REFERENCES
[1] | Calabresi P , Ghiglieri V , Mazzocchetti P , Corbelli I , Picconi B ((2015) ) Levodopa-induced plasticity: A double-edged sword in Parkinson’s disease? Proc R Soc Lond B Biol Sci 370: , 20140184. |
[2] | Bogetofte H , Alamyar A , Blaabjerg M , Meyer M ((2020) ) Levodopa therapy for Parkinson’s disease: History, current status and perspectives. CNS Neurol Disord 19: , 572–583. |
[3] | Santini E ((2009) ) Molecular basis of L-DOPA-induced dyskinesia: Studies on striatal signaling, Karolinska Institutet, Sweden. |
[4] | Bastide MF , Meissner WG , Picconi B , Fasano S , Fernagut P-O , Feyder M , Francardo V , Alcacer C , Ding Y , Brambilla R ((2015) ) Pathophysiology of L-dopa-induced motor and non-motor complications in Parkinson’s disease. Prog Neurobiol 132: , 96–168. |
[5] | de la Fuente-Fernández R , Sossi V , Huang Z , Furtado S , Lu J-Q , Calne DB , Ruth TJ , Stoessl AJ ((2004) ) Levodopa-induced changes in synaptic dopamine levels increase with progression of Parkinson’s disease: implications for dyskinesias. Brain 127: , 2747–2754. |
[6] | Lindgren HS , Andersson DR , Lagerkvist S , Nissbrandt H , Cenci MA ((2010) ) l-DOPA-induced dopamine efflux in the striatum and the substantia nigra in a rat model of Parkinson’s disease: Temporal and quantitative relationship to the expression of dyskinesia. J Neurochem 112: , 1465–1476. |
[7] | Jenner P ((2008) ) Molecular mechanisms of L-DOPA-induced dyskinesia. Nat Rev Neurosci 9: , 665–677. |
[8] | Cotzias GC , Van Woert MH , Schiffer LM ((1967) ) Aromatic amino acids and modification of parkinsonism. New Engl J Med 276: , 374–379. |
[9] | Carta M , Carlsson T , Muñoz A , Kirik D , Björklund A ((2008) ) Involvement of the serotonin system in L-dopa-induced dyskinesias. Parkinsonism Relat Disord 14: , S154–S158. |
[10] | Gil S , Park C , Lee J , Koh H ((2010) ) The roles of striatal serotonin and l-amino-acid decarboxylase on l-DOPA-induced dyskinesia in a hemiparkinsonian rat model. Cell Mol Neurobiol 30: , 817–825. |
[11] | Lanza K , Bishop C ((2018) ) Serotonergic targets for the treatment of L-DOPA-induced dyskinesia. J Neural Transm 125: , 1203–1216. |
[12] | Carta M , Carlsson T , Kirik D , Björklund A ((2007) ) Dopamine released from 5-HT terminals is the cause of L-DOPA-induced dyskinesia in parkinsonian rats. Brain 130: , 1819–1833. |
[13] | Eskow KL , Gupta V , Alam S , Park JY , Bishop C ((2007) ) The partial 5-HT1A agonist buspirone reduces the expression and development of l-DOPA-induced dyskinesia in rats and improves l-DOPA efficacy. Pharmacol Biochem Behav 87: , 306–314. |
[14] | Dekundy A , Lundblad M , Danysz W , Cenci MA ((2007) ) Modulation of L-DOPA-induced abnormal involuntary movements by clinically tested compounds: further validation of the rat dyskinesia model. Behav Brain Res 179: , 76–89. |
[15] | Paolone G , Brugnoli A , Arcuri L , Mercatelli D , Morari M ((2015) ) Eltoprazine prevents levodopa-induced dyskinesias by reducing striatal glutamate and direct pathway activity. Mov Disord 30: , 1728–1738. |
[16] | Durif F , Debilly B , Galitzky M , Morand D , Viallet F , Borg M , Thobois S , Broussolle E , Rascol O ((2004) ) Clozapine improves dyskinesias in Parkinson disease: A double-blind, placebo-controlled study. Neurology 62: , 381–388. |
[17] | Bishop C , George JA , Buchta W , Goldenberg AA , Mohamed M , Dickinson SO , Eissa S , Eskow Jaunarajs KL ((2012) ) Serotonin transporter inhibition attenuates l-DOPA-induced dyskinesia without compromising l-DOPA efficacy in hemi-parkinsonian rats. Eur J Neurosci 36: , 2839–2848. |
[18] | Mazzucchi S , Frosini D , Ripoli A , Nicoletti V , Linsalata G , Bonuccelli U , Ceravolo R ((2015) ) Serotonergic antidepressant drugs and L-dopa-induced dyskinesias in Parkinson’s disease. Acta Neurol Scand 131: , 191–195. |
[19] | Olanow CW , Damier P , Goetz CG , Mueller T , Nutt J , Rascol O , Serbanescu A , Deckers F , Russ H ((2004) ) Multicenter, open-label, trial of sarizotan in Parkinson disease patients with levodopa-induced dyskinesias (the SPLENDID Study). Clin Neuropharmacol 27: , 58–62. |
[20] | Svenningsson P , Rosenblad C , af Edholm Arvidsson K , Wictorin K , Keywood C , Shankar B , Lowe DA , Björklund A , Widner H ((2015) ) Eltoprazine counteracts l-DOPA-induced dyskinesias in Parkinson’s disease: A dose-finding study. Brain 138: , 963–973. |
[21] | Lindenbach D , Palumbo N , Ostock CY , Vilceus N , Conti MM , Bishop C ((2015) ) Side effect profile of 5-HT treatments for P arkinson’s disease and L-DOPA-induced dyskinesia in rats. Br J Pharmacol 172: , 119–130. |
[22] | Corsi S , Stancampiano R , Carta M ((2021) ) Serotonin/dopamine interaction in the induction and maintenance of L-DOPA-induced dyskinesia: An update. Prog Brain Res 261: , 287–302. |
[23] | Nakatani Y , Sato-Suzuki I , Tsujino N , Nakasato A , Seki Y , Fumoto M , Arita H ((2008) ) Augmented brain 5-HT crosses the blood-brain barrier through the 5-HT transporter in rat. Eur J Neurosci 27: , 2466–2472. |
[24] | Tronci E , Lisci C , Stancampiano R , Fidalgo C , Collu M , Devoto P , Carta M ((2013) ) 5-Hydroxy-tryptophan for the treatment of L-DOPA-induced dyskinesia in the rat Parkinson’s disease model. Neurobiol Dis 60: , 108–114. |
[25] | Meloni M , Puligheddu M , Sanna F , Cannas A , Farris R , Tronci E , Figorilli M , Defazio G , Carta M ((2020) ) Efficacy and safety of 5-Hydroxytryptophan on levodopa-induced motor complications in Parkinson’s disease: A preliminary finding. J Neurol Sci 415: , 116869. |
[26] | Tseng K-Y , Kuo T-T , Wang V , Huang EY-K , Ma K-H , Olson L , Hoffer BJ , Chen Y-H ((2022) ) Tetrabenazine mitigates aberrant release and clearance of dopamine in the nigrostriatal system, and alleviates L-DOPA-induced dyskinesia in a mouse model of Parkinson’s disease. J Parkinsons Dis 12: , 1545–1565. |
[27] | Rubinow DR , Schmidt PJ , Roca CA ((1998) ) Estrogen–serotonin interactions: Implications for affective regulation. Biol Psychiatry 44: , 839–850. |
[28] | Thibeault A-AH , Sanderson JT , Vaillancourt C ((2019) ) Serotonin-estrogen interactions: What can we learn from pregnancy? Biochimie 161: , 88–108. |
[29] | Jurado-Coronel JC , Cabezas R , Rodríguez MFÁ , Echeverria V , García-Segura LM , Barreto GE ((2018) ) Sex differences in Parkinson’s disease: Features on clinical symptoms, treatment outcome, sexual hormones and genetics. Front Neuroendocrinol 50: , 18–30. |
[30] | Kolmančič K , Živin M , Zorović M ((2022) ) Modulation by estradiol of L-dopa-induced dyskinesia in a rat model of post-menopausal hemiparkinsonism. Life 12: , 640. |
[31] | Quinn NP , Marsden CD ((1986) ) Menstrual-related fluctuations in Parkinson’s disease. Mov Disord 1: , 85–87. |
[32] | Ekstrand MI , Terzioglu M , Galter D , Zhu S , Hofstetter C , Lindqvist E , Thams S , Bergstrand A , Hansson FS , Trifunovic A , Hoffer B , Cullheim S , Mohammed AH , Olson L , Larsson NG ((2007) ) Progressive parkinsonism in mice with respiratory-chain-deficient dopamine neurons. Proc Natl Acad Sci U S A 104: , 1325–1330. |
[33] | Galter D , Pernold K , Yoshitake T , Lindqvist E , Hoffer B , Kehr J , Larsson NG , Olson L ((2010) ) MitoPark mice mirror the slow progression of key symptoms and L-DOPA response in Parkinson’s disease. Genes Brain Behav 9: , 173–181. |
[34] | Good CH , Hoffman AF , Hoffer BJ , Chefer VI , Shippenberg TS , Backman CM , Larsson NG , Olson L , Gellhaar S , Galter D , Lupica CR ((2011) ) Impaired nigrostriatal function precedes behavioral deficits in a genetic mitochondrial model of Parkinson’s disease. FASEB J 25: , 1333–1344. |
[35] | Ding Y , Restrepo J , Won L , Hwang DY , Kim KS , Kang UJ ((2007) ) Chronic 3,4-dihydroxyphenylalanine treatment induces dyskinesia in aphakia mice, a novel genetic model of Parkinson’s disease. Neurobiol Dis 27: , 11–23. |
[36] | Sebastianutto I , Maslava N , Hopkins CR , Cenci MA ((2016) ) Validation of an improved scale for rating l-DOPA-induced dyskinesia in the mouse and effects of specific dopamine receptor antagonists. Neurobiol Dis 96: , 156–170. |
[37] | Ma K-H , Huang W-S , Kuo Y-Y , Peng C-J , Liou N-H , Liu R-S , Hwang J-J , Liu J-C , Chen H-J , Shiue C-Y ((2009) ) Validation of 4-[18F]-ADAM as a SERT imaging agent using micro-PET and autoradiography. Neuroimage 45: , 687–693. |
[38] | Li IH , Huang W-S , Shiue C-Y , Huang Y-Y , Liu R-S , Chyueh S-C , Hu S-H , Liao M-H , Shen L-H , Liu J-C ((2010) ) Study on the neuroprotective effect of fluoxetine against MDMA-induced neurotoxicity on the serotonin transporter in rat brain using micro-PET. NeuroImage 49: , 1259–1270. |
[39] | Chen YH , Harvey BK , Hoffman AF , Wang Y , Chiang YH , Lupica CR ((2008) ) MPTP-induced deficits in striatal synaptic plasticity are prevented by glial cell line-derived neurotrophic factor expressed via an adeno-associated viral vector. FASEB J 22: , 261–275. |
[40] | Good CH , Wang H , Chen YH , Mejias-Aponte CA , Hoffman AF , Lupica CR ((2013) ) Dopamine D4 receptor excitation of lateral habenula neurons via multiple cellular mechanisms. J Neurosci 33: , 16853–16864. |
[41] | Chen Y-H , Huang EY-K , Kuo T-T , Hoffer BJ , Miller J , Chou Y-C , Chiang Y-H ((2017) ) Dopamine release in the nucleus accumbens is altered following traumatic brain injury. Neuroscience 348: , 180–190. |
[42] | Cho H-Y , Reddy SP , Kleeberger SR ((2006) ) Nrf2 defends the lung from oxidative stress. Antioxid Redox Signal 8: , 76–87. |
[43] | Kawagoe KT , Zimmerman JB , Wightman RM ((1993) ) Principles of voltammetry and microelectrode surface states. J Neurosci Methods 48: , 225–240. |
[44] | Wu L-Y , Chen J-F , Tao P-L , Huang EY-K ((2009) ) Attenuation by dextromethorphan on the higher liability to morphine-induced reward, caused by prenatal exposure of morphine in rat offspring. J Biomed Sci 16: , 1–11. |
[45] | Santini E , Valjent E , Usiello A , Carta M , Borgkvist A , Girault J-A , Hervé D , Greengard P , Fisone G ((2007) ) Critical involvement of cAMP/DARPP-32 and extracellular signal-regulated protein kinase signaling in L-DOPA-induced dyskinesia. J Neurosci 27: , 6995–7005. |
[46] | Politis M , Wu K , Loane C , Brooks DJ , Kiferle L , Turkheimer FE , Bain P , Molloy S , Piccini P ((2014) ) Serotonergic mechanisms responsible for levodopa-induced dyskinesias in Parkinson’s disease patients. J Clin Invest 124: , 1340–1349. |
[47] | Rylander D , Parent M , O’Sullivan SS , Dovero S , Lees AJ , Bezard E , Descarries L , Cenci MA ((2010) ) Maladaptive plasticity of serotonin axon terminals in levodopa-induced dyskinesia. Ann Neurol 68: , 619–628. |
[48] | Larsen MB , Sonders MS , Mortensen OV , Larson GA , Zahniser NR , Amara SG ((2011) ) Dopamine transport by the serotonin transporter: A mechanistically distinct mode of substrate translocation. J Neurosci 31: , 6605–6615. |
[49] | Conti Mazza MM , Centner A , Werner DF , Bishop C ((2023) ) Striatal serotonin transporter gain-of-function in L-DOPA-treated, hemi-parkinsonian rats. Brain Res 1811: , 148381. |
[50] | Cenci MA ((2007) ) Dopamine dysregulation of movement control in L-DOPA-induced dyskinesia. Trends Neurosci 30: , 236–243. |
[51] | Carta M , Carlsson T , Muñoz A , Kirik D , Björklund A ((2008) ) Serotonin–dopamine interaction in the induction and maintenance of L-DOPA-induced dyskinesias. In Serotonin-Dopamine Interaction: Experimental Evidence and Therapeutic Relevance, Di Giovanni G, Di Matteo V, Esposito E, eds. Elsevier, Amsterdam, pp. 465–478. |
[52] | Carlsson T , Carta M , Winkler C , Björklund A , Kirik D ((2007) ) Serotonin neuron transplants exacerbate L-DOPA-induced dyskinesias in a rat model of Parkinson’s disease. J Neurosci 27: , 8011–8022. |
[53] | Muñoz A , Carlsson T , Tronci E , Kirik D , Björklund A , Carta M ((2009) ) Serotonin neuron-dependent and-independent reduction of dyskinesia by 5-HT1A and 5-HT1B receptor agonists in the rat Parkinson model. Exp Neurol 219: , 298–307. |
[54] | Zeng B-Y , Iravani MM , Jackson MJ , Rose S , Parent A , Jenner P ((2010) ) Morphological changes in serotoninergic neurites in the striatum and globus pallidus in levodopa primed MPTP treated common marmosets with dyskinesia. Neurobiol Dis 40: , 599–607. |
[55] | Wang V , Kuo TT , Huang EY , Ma KH , Chou YC , Fu ZY , Lai LW , Jung J , Choi HI , Choi DS , Li Y , Olson L , Greig NH , Hoffer BJ , Chen YH ((2021) ) Sustained release GLP-1 agonist PT320 delays disease progression in a mouse model of Parkinson’s disease. ACS Pharmacol Transl Sci 4: , 858–869. |
[56] | Wang V , Tseng KY , Kuo TT , Huang EY , Lan KL , Chen ZR , Ma KH , Greig NH , Jung J , Choi HI , Olson L , Hoffer BJ , Chen YH ((2024) ) Attenuating mitochondrial dysfunction and morphological disruption with PT320 delays dopamine degeneration in MitoPark mice. J Biomed Sci 31: , 38. |
[57] | Tronci E , Fidalgo C , Stancampiano R , Carta M ((2015) ) Effect of selective and non-selective serotonin receptor activation on l-DOPA-induced therapeutic efficacy and dyskinesia in parkinsonian rats. Behav Brain Res 292: , 300–304. |
[58] | Munoz A , Li Q , Gardoni F , Marcello E , Qin C , Carlsson T , Kirik D , Di Luca M , Björklund A , Bezard E ((2008) ) Combined 5-HT1A and 5-HT1B receptor agonists for the treatment of L-DOPA-induced dyskinesia. Brain 131: , 3380–3394. |
[59] | Choi Y , Huh E , Lee S , Kim JH , Park MG , Seo S-Y , Kim SY , Oh MS ((2023) ) 5-hydroxytryptophan reduces levodopa-induced dyskinesia via regulating AKT/mTOR/S6K and CREB/ΔFosB signals in a mouse model of Parkinson’s disease. Biomol Ther (Seoul) 31: , 402. |
[60] | Fidalgo C , Ko WKD , Tronci E , Li Q , Stancampiano R , Chuan Q , Bezard E , Carta M ((2015) ) Effect of serotonin transporter blockade on L-DOPA-induced dyskinesia in animal models of Parkinson’s disease. Neuroscience 298: , 389–396. |
[61] | Conti MM , Ostock CY , Lindenbach D , Goldenberg AA , Kampton E , Dell’isola R , Katzman AC , Bishop C ((2014) ) Effects of prolonged selective serotonin reuptake inhibition on the development and expression of L-DOPA-induced dyskinesia in hemi-parkinsonian rats. Neuropharmacology 77: , 1–8. |
[62] | Kuan W-L , Zhao J-W , Barker RA ((2008) ) The role of anxiety in the development of levodopa-induced dyskinesias in an animal model of Parkinson’s disease, and the effect of chronic treatment with the selective serotonin reuptake inhibitor citalopram. Psychopharmacology 197: , 279–293. |
[63] | Malagié I , Trillat A-C , Jacquot C , Gardier AM ((1995) ) Effects of acute fluoxetine on extracellular serotonin levels in the raphe: An in vivo microdialysis study. Eur J Pharmacol 286: , 213–217. |
[64] | Blier P , Bergeron R , de Montigny C ((1997) ) Selective activation of postsynaptic 5-HT1A receptors induces rapid antidepressant response. Neuropsychopharmacology 16: , 333–338. |
[65] | Casanovas JM , Lesourd M , Artigas F ((1997) ) The effect of the selective 5-HT1A agonists alnespirone (S-20499) and 8-OH-DPAT on extracellular 5-hydroxytryptamine in different regions of rat brain. Br J Pharmacol 122: , 733–741. |