Effects of xenon gas on human airway epithelial cells during hyperoxia and hypothermia
Abstract
BACKGROUND:
Hypothermia with xenon gas has been used to reduce brain injury and disability rate after perinatal hypoxia-ischemia. We evaluated xenon gas therapy effects in an in vitro model with or without hypothermia on cultured human airway epithelial cells (Calu-3).
METHODS:
Calu-3 monolayers were grown at an air-liquid interface and exposed to one of the following conditions: 1) 21% FiO2 at 37°C (control); 2) 45% FiO2 and 50% xenon at 37°C; 3) 21% FiO2 and 50% xenon at 32°C; 4) 45% FiO2 and 50% xenon at 32°C for 24 hours. Transepithelial resistance (TER) measurements were performed and apical surface fluids were collected and assayed for total protein, IL-6, and IL-8. Three monolayers were used for immunofluorescence localization of zonula occludens-1 (ZO-1). The data were analyzed by one-way ANOVA.
RESULTS:
TER decreased at 24 hours in all treatment groups. Xenon with hyperoxia and hypothermia resulted in greatest decrease in TER compared with other groups. Immunofluorescence localization of ZO-1 (XY) showed reduced density of ZO-1 rings and incomplete ring-like staining in the 45% FiO2– 50% xenon group at 32°C compared with other groups. Secretion of total protein was not different among groups. Secretion of IL-6 in 21% FiO2 with xenon group at 32°C was less than that of the control group. The secretion of IL-8 in 45% FiO2 with xenon at 32°C was greater than that of other groups.
CONCLUSION:
Hyperoxia and hypothermia result in detrimental epithelial cell function and inflammation over 24-hour exposure. Xenon gas did not affect cell function or reduce inflammation.
1Introduction
Perinatal hypoxic-ischemic brain injury occurs in one to six neonates every 1000 live births. Despite remarkable advances in medicine, the rate of perinatal mortality of neonates with hypoxic-ischemic brain injury remains at 15% to 20% [1–5]. In recent years, therapeutic hypothermia has shown beneficial effects in animal hypoxic-ischemic models and clinical trials of either selective head cooling with mild systemic hypothermia [6] or total body cooling [7, 8]. In animal hypoxia-ischemia models, hypothermia (rectal temperature of 33.5°C) has shown promising neuroprotection [9–12]. Clinical studies show that hypothermia within 24 hours of birth in neonates reduced death and disability from 66% to 50% [11, 13–15]. However, a meta-analysis revealed that moderate hypothermia significantly increased the risk of thrombocytopenia and cardiac arrhythmia [7], which limited its application in the preterm infant [8, 16]. To further reduce brain injury and the rate of disability after perinatal hypoxia-ischemia events, it is important to explore new therapies. One recent therapeutic intervention in perinatal hypoxic-ischemic brain injury is the use of hypothermia induction with inhalation of xenon gas via a closed-circuit neonatal xenon delivery system [17]. Hypothermia with xenon gas has been used to treat newborn infants with hypoxic-ischemic encephalopathy to reduce brain damage and long-term complications. The combination of hypothermia and xenon gas offers better neuro protection than xenon gas or hypothermia alone, evident by global histological neuro protection, as well as improved regional neuro protection [18]. Xenon, a noble gas, is a noncompetitive inhibitor of the N-methyl D-aspartate (NMDA) glutamate receptor in the brain and an anti-apoptotic agent [18–20]. Most importantly, xenon has great water solubility and a higher fat-water solubility ratio [8, 21]. When inhaled, xenon can be absorbed effectively into the system, and it crosses the blood–brain barrier and diffuses into injury areas of the central nervous system rapidly. In 1939, its anesthetic properties were recognized [22]. Stable xenon is radio dense and has been used to enhance computed tomography (xenon/CT) since the late 1970s [23, 24]. There are no allergies, toxicities, adverse hemodynamic clinical side effects, or renal effects associated with xenon inhalation [15, 16, 18, 25–27]. The effects of xenon gas are dependent upon its concentration [19, 28, 29]. Alveolar xenon concentrations of 50% have proven neuro protective in vitro and in vivo in the rat hypoxia model; alveolar concentration of 60% to 70% xenon acts as an anesthetic in humans [16, 30], and 80% xenon inhibits the maximum NMDA receptor [21, 31, 32]. Furthermore, xenon prevents secondary cellular damage after primary neuron damage by reducing the over-release of neurotransmitters [6, 33]. In addition, early intervention with 30% to 50% inhaled xenon and moderate whole-body hypothermia for 3 to 24 hours in neonates with hypoxic-ischemic brain injury [31, 32] showed anticonvulsant effects and long-term additive neuro protection [21, 31].
While the effects of both localized hypothermia and inspired xenon gas are documented in regard to preventing brain damage in neonates experiencing hypoxic-ischemic events (in the form of clinical and animal studies), little is known regarding the pulmonary effects of xenon with or without hyperoxia on human airway epithelium during inhalation treatment. In this study, we sought to evaluate the effects of xenon gas therapy in an in vitro model with or without hypothermia on cultured human airway epithelial cells (Calu-3). In this regard, we hypothesized that the combination of xenon and hypothermia would not have a deleterious effect on lung cell function, biochemical response, or structure.
2Materials and methods
2.1Calu-3 cell culture
As previously described, cell culture reagents were purchased from Invitrogen (Carlsbad, CA). Transwell permeable supports (0.4μm polyester membrane) were purchased from Corning Incorporated Life Sciences (Acton, MA). Calu-3 cells were cultured at 37°C and 5% CO2 in a 50% /50% mixture of Dulbecco’s modified Eagle’s medium/Ham’s F-12 (DMEM/F12) supplemented with 13% fetal bovine serum, 500 u/ml penicillin, and 50μg/ml streptomycin. Calu-3 cells were grown in 75 cm2 tissue culture flasks and split when 90% to 95% confluent.
Calu-3 cell air-liquid interface culturing was carried out as previously described [34]. For the present study, Calu-3 cells were plated at 2×106 cells/mL onto Costar transwell inserts (0.4μm pore size, 12 mm diameter, clear polycarbonate membrane; Costar plate; Corning, Acton, MA) that had been coated with human type I collagen (Southern Biotech, Birmingham, AL). Apical culture medium was removed on the second day after plating, and monolayers were grown at an air-liquid interface by feeding the cells from the basolateral side with DMEM/F12 with 13% fetal bovine serum, and the apical side was exposed to air. The medium on the basolateral side was changed on alternate days.
After 11 days of transwell culture, full confluence was verified by measurement of transepithelial resistance (TER) with STX2 electrodes and an epithelial volt ohm meter (World Precision Instruments, Sarasota, FL). Calu-3 monolayers that exhibited TER values of at least 800 to 1,000 ohm·cm2 were exposed to one of the following conditions in a modified modular incubator chamber (MIC-101; Billups-Rothenberg, Del Mar, CA): 1) 21% FiO2 at 37°C (normoxia and normothermia); 2) 21% FiO2 and 50% xenon at 32°C (normoxia and hypothermia with xenon); 3) 45% FiO2 and 50% xenon at 37°C (hyperoxia and normothermia with xenon); or 4) 45% FiO2 and 50% xenon at 32°C (hyperoxia and hypothermia with xenon) for 24 hours (Fig. 1). To achieve the desired gas concentrations listed above, gases containing 21% FiO2 (FiO2 = 21%, FiCO2 = 5%, balance nitrogen) and 95% FiO2 (FiO2 = 95%, FiCO2 = 5%) were blended using a Servo O2-air 960 mixer (Siemens-Elema, Sweden), then the blended gas and 100% xenon were connected with a Cole-Parmer Flow meter (Cole-Parmer, Vernon Hills, IL). The appropriate gases were conditioned to 32°C or 37°C, humidified with an MR 730 respiratory humidifier (Fisher & Paykel Healthcare, Laguna Hills, CA), and then flushed through the airtight chamber at a flow rate of 5 L/min for 10 minutes. The chamber oxygen level was monitored with an oxygen analyzer (MAXO2) (OM-25AE; Maxtec, Salt Lake City, UT). After purging, the chamber was disconnected from the gas source and sealed. To avoid pressurizing the chamber, the inlet clamp was closed first, then the outlet clamp. The chamber was placed in an incubator at 32°C or 37°C for 24 hours.
Fig. 1
Calu-3 monolayers and transwell insert. Calu-3 cell monolayers were grown at an air-liquid interface to full confluence before exposure to one of four conditions as described above.
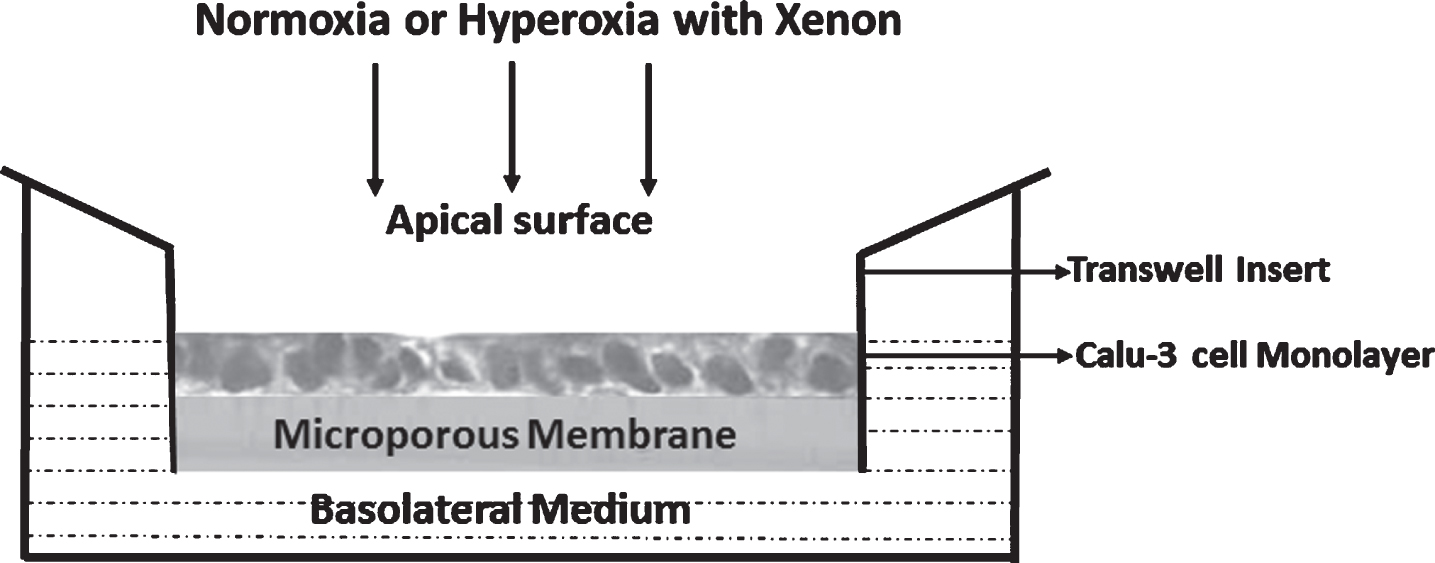
2.2Collection of Calu-3 apical surface fluid washings (ASF)
In each of the experimental conditions, Calu-3 ASF was collected from six monolayers for each condition at 24 hours. As previously described [35], the apical surface of monolayer was washed twice with 140μl of normal saline, which was combined for a total of 280μl per sample. Samples were centrifuged for 15 minutes at 13,000 rcf and 4°C to remove the cellular debris, and the supernatants were stored in aliquots at – 70°C for subsequent total protein, interleukin (IL)-6, and IL-8 assays.
2.3Measurement of TER
As previously described [35], TER of Calu-3 monolayers was measured using STX2 electrodes and an epithelial volt ohm meter. At baseline and after treatment, 0.5 mL of medium was added apically, 1 mL of medium was added basolaterally, and electrodes were inserted into each pool of medium to measure TER for each Calu-3 monolayer. The resistance of four transwell inserts without Calu-3 monolayers was measured as control.
2.4Immunofluorescence localization of ZO-1 (XY) and confocal microscopy
Four monolayers were used for immunefluorescence localization of zonula occludens-1 (ZO-1) using permeabilized technique (cold methanol fixation) [36]. Monolayers were washed once using phosphate-buffered saline (PBS) with 100μM CaCl2–1 mM MgCl2 (CM) and fixed with cold methanol (–20°C) for 20 min at –20°C. The methanol was aspirated and the monolayers were rehydrated with PBS-CM-bovine serum albumin (BSA) three times for 10 minutes each. The monolayers were incubated with primary antibody anti-ZO-1 (1 : 100) and PBS (negative control) overnight at 4°C. The next day, the monolayers were washed again three times for 10 minutes each with PBS-CM-BSA then incubated with the secondary antibody, Fluorescein (FITC)-AffiniPure Goat Anti-Rabbit IgG (H + L) (Jackson Immuno Research, West Grove, PA) (1 : 200), for 1 hour in a humidified chamber. Monolayers were washed, cut from the transwell inserts, and mounted in a Vectashield (Vector Laboratories, Burlingame, CA).
Tight junction images were captured and viewed with a Leica SP-2 confocal microscope (Leica, Oberkochen, Germany). The images were obtained either by a single scan with averages (if the image was considered level enough) or by a series scan with image averages.
2.5Measurement of total protein in Calu-3 ASF
Total protein concentration of Calu-3 ASF was measured in duplicate using a DC protein assay kit (Bio-Rad, Hercules, CA). BSA (standard II) was selected as a reference protein standard at 3–5 dilutions from 0.2 mg/ml to about 1.5 mg/ml protein. Absorbance was read at 655 nm.
2.6Measurement of IL-6 and IL-8 level in Calu-3 ASF
The levels of IL-6 and IL-8 in Calu-3 ASF were measured with quantitative enzyme-linked immunosorbent assay (ELISA) using human IL-6 and IL-8 Quantikine ELISA kits (R & D Systems, Minneapolis, MN). Calu-3 ASF washings used in the assay were appropriately diluted. All standards and samples were assayed in duplicate. The test sensitivity for respective immunoassays was as follows: IL-6 (0.039 pg/ml) and IL-8 (10 pg/ml). Inter-assay and intra-assay coefficients of variance are less than 10%.
2.7Statistical analysis
After 11 days of air-liquid interface culturing, Calu-3 cells developed a stable baseline TER as shown previously [35] for all monolayers prior to hyperoxic exposure. At 24 hours, summarized data were presented as mean and standard error (SEM). On the basis of a number of our epithelial airway studies, [35, 37–40] to mention a few, we have found that our cell preparations of 6–8 samples/group gave significant results and variances with respect to our primary outcomes and supported earlier power analyses. Differences among conditions for each parameter compared with control samples were analyzed using one-way analyses of variance (ANOVA) at 24 hours, and post-hoc analyses were done using Bonferroni correction. All tests were compared with a level of significance of p < 0.05. Statistical software SPSS version 23 (IBM, Armonk, NY) and Excel 2016 (Microsoft, Redmond, WA) were used to perform the statistical analysis and data plotting.
3Results
3.1Measurement of TER
Transepithelial resistance data are shown in Fig. 2. After 11 days in culture, Calu-3 cells developed a baseline TER of 1026.4±59 ohm·cm2for all monolayers prior to hyperoxic exposure. At 24 hours, a one-way ANOVA revealed that TER decreased in all treatment groups. There was a difference between control (21% FiO2–37°C) and other treatment groups (p < 0.001); at 32°C, the decrease in TER was greater for hyperoxia and xenon than normoxia and xenon (p < 0.05).
Fig. 2
TER (% decrease) for Calu-3 monolayers exposed to one of four conditions as described above. TER decreased at 24 hours in all treatment groups. There was difference between control and other treatment groups (p < 0.001); at 32°C, there was difference between xenon with normoxia and xenon with hyperoxia group (p < 0.05). Data are mean±SEM. *Group effect (p < 0.05). N = 6 for each condition. Xe = xenon.
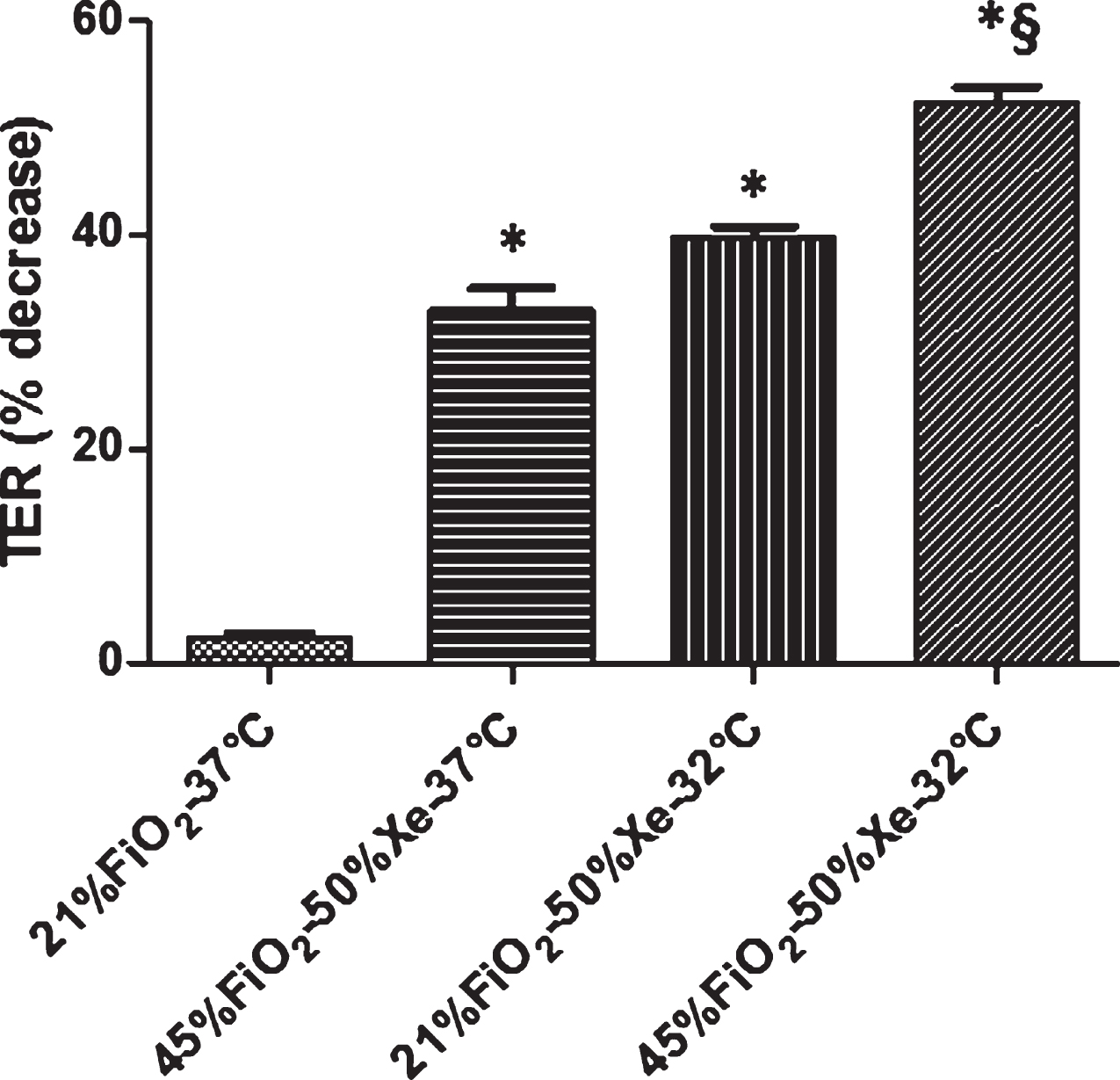
3.2Immunofluorescence localization of ZO-1 (XY)
Immunofluorescence localization of ZO-1 showed incomplete and reduced density of the ZO-1 rings in room air and hyperoxia with hypothermia groups at 24 hours compared with control group (Fig. 3).
Fig. 3
Representative tight junction images. Immunofluorescence localization of ZO-1 (XY) showed reduced density of the ZO-1 rings and the incomplete ring-like staining in the 45% FiO2-50% xenon group at 32°C compared with other groups. The intensity of the cytoplasm in this group was greater than that in the other groups.
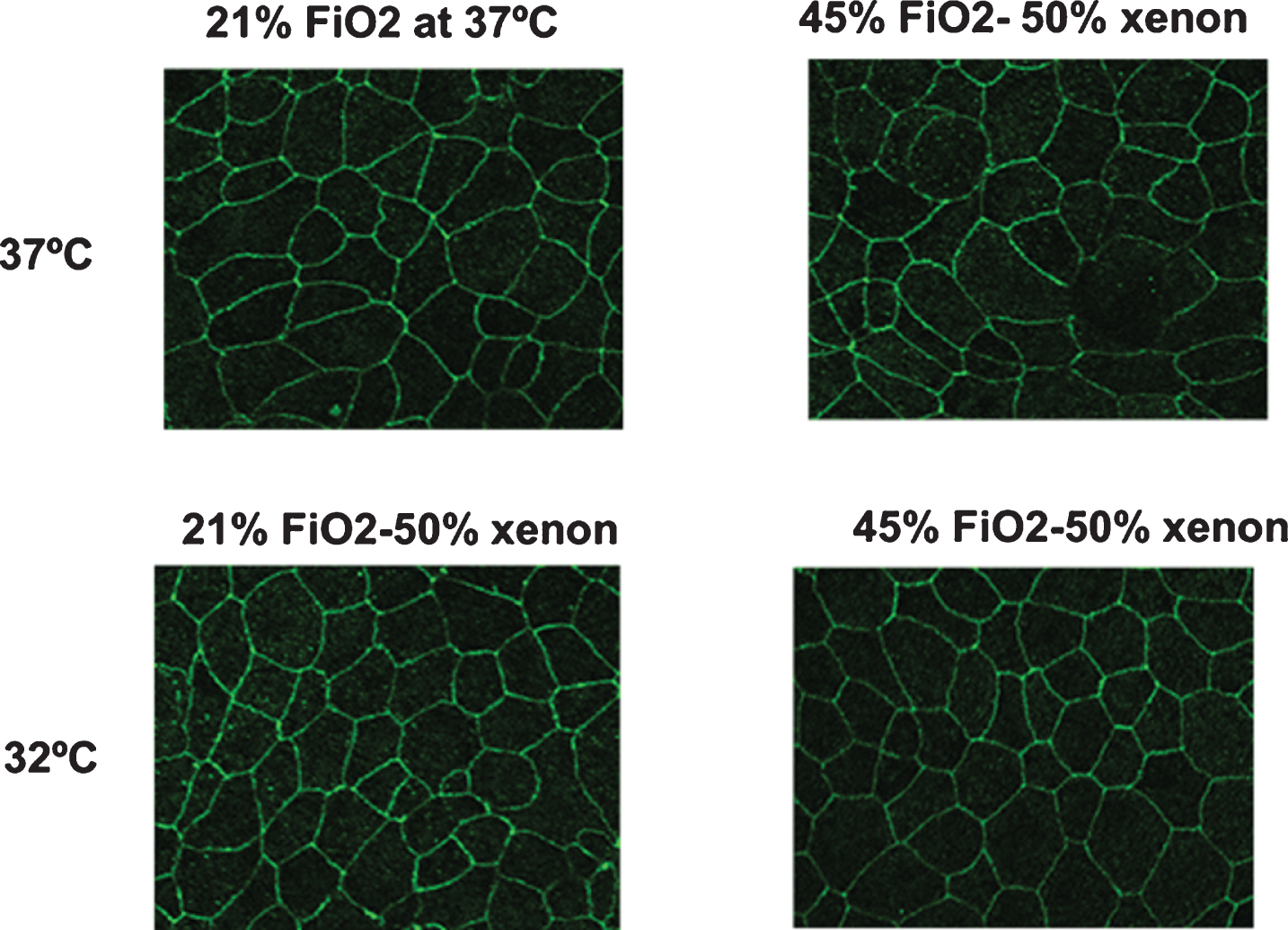
3.3Measurement of total protein in Calu-3 ASF
Data for ASF total protein concentration from monolayers are presented in Fig. 4. Secretion of total protein was not different among groups (p > 0.05).
Fig. 4
The secretion of total protein in ASF from Calu-3 monolayers. There was no difference among groups at 24 hours (p > 0.05). Data are mean±SEM (N = 6). Xe = xenon.
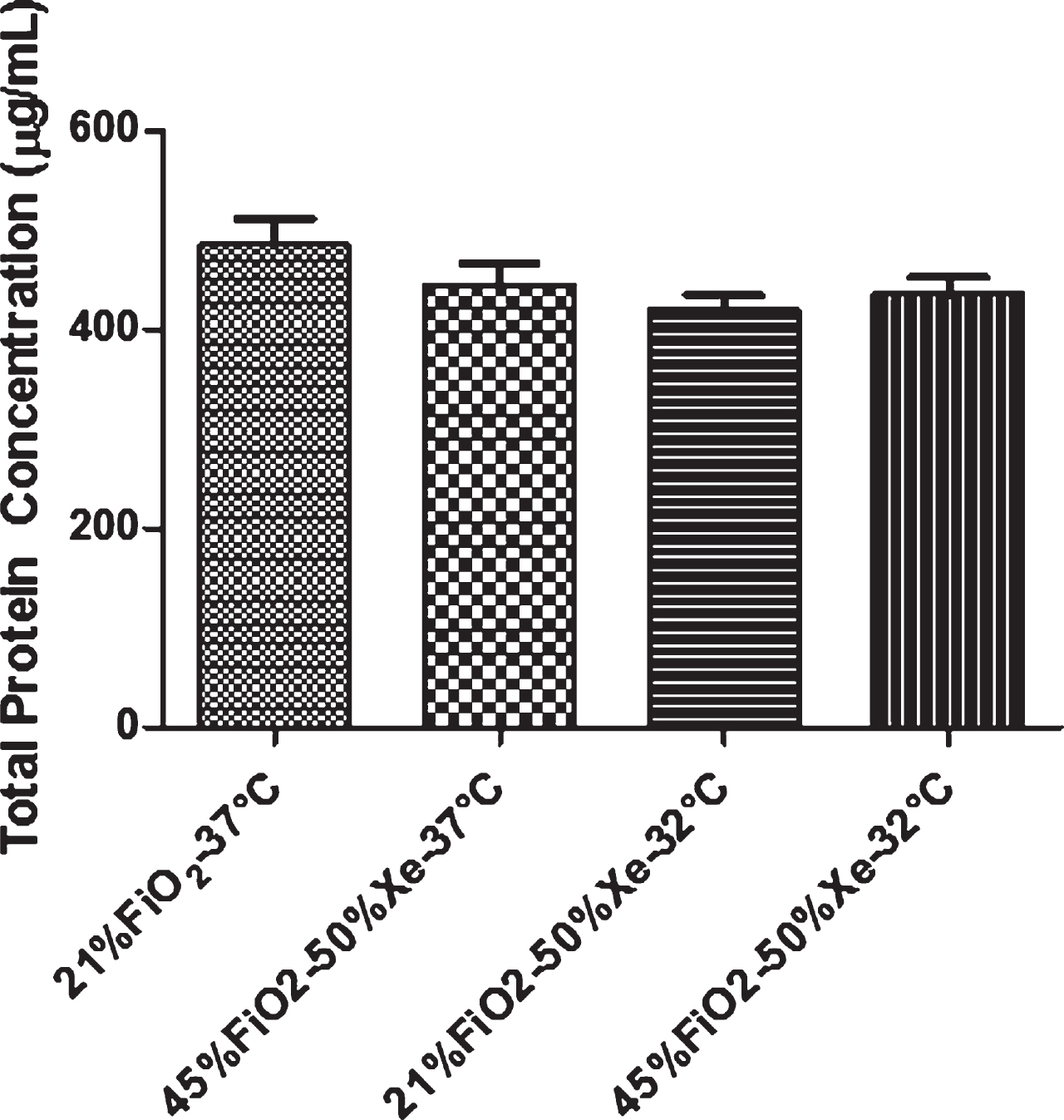
3.4Measurement of IL-6 and IL-8 level in Calu-3 ASF
Data for ASF IL-6 concentration from monolayers exposed to different conditions are presented in Fig. 5. Secretion of IL-6 in 21% FiO2/xenon group at 32°C was less than that of the control group (p < 0.05). Data for ASF IL-8 concentration are presented in Fig. 6. The secretion of IL-8 in 45% FiO2/xenon at 32°C was greater than that of the 45% FiO2/xenon group at 37°C and 21% FiO2/xenon group at 32°C (p < 0.05).
Fig. 5
Pro-inflammatory cytokines in ASF from Calu-3 monolayers. Secretion of IL-6 in 21% FiO2/xenon group at 32°C was less than that of the control group (p < 0.05). Data are mean±SEM (N = 6). Xe = xenon.
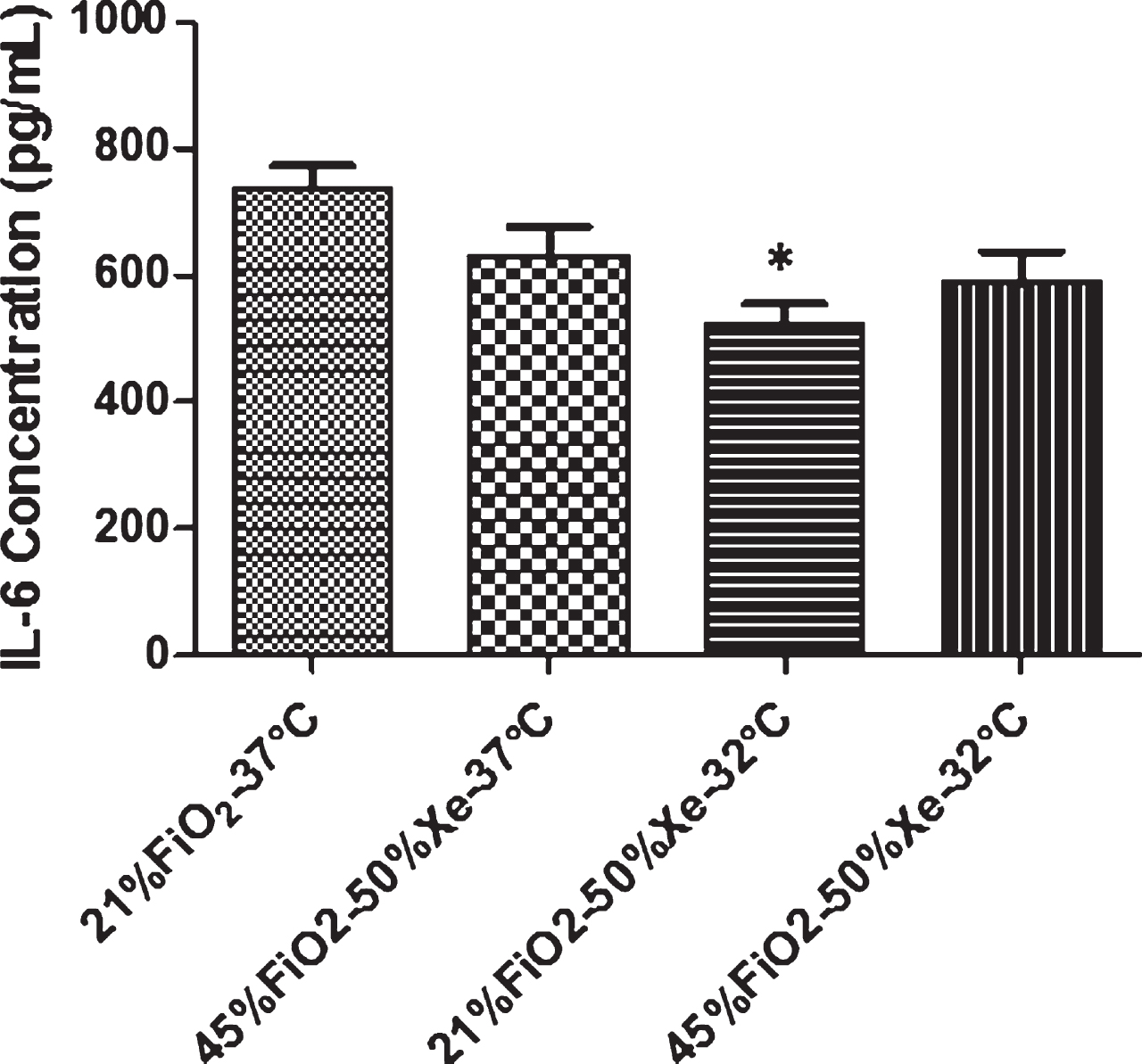
Fig. 6
Pro-inflammatory cytokines in ASF from Calu-3 monolayers. The secretion of IL-8 in 45% FiO2/50% xenon at 32°C was greater than that of other groups (p < 0.05). *Group effect. Data are mean±SEM (N = 6). Xe = xenon.
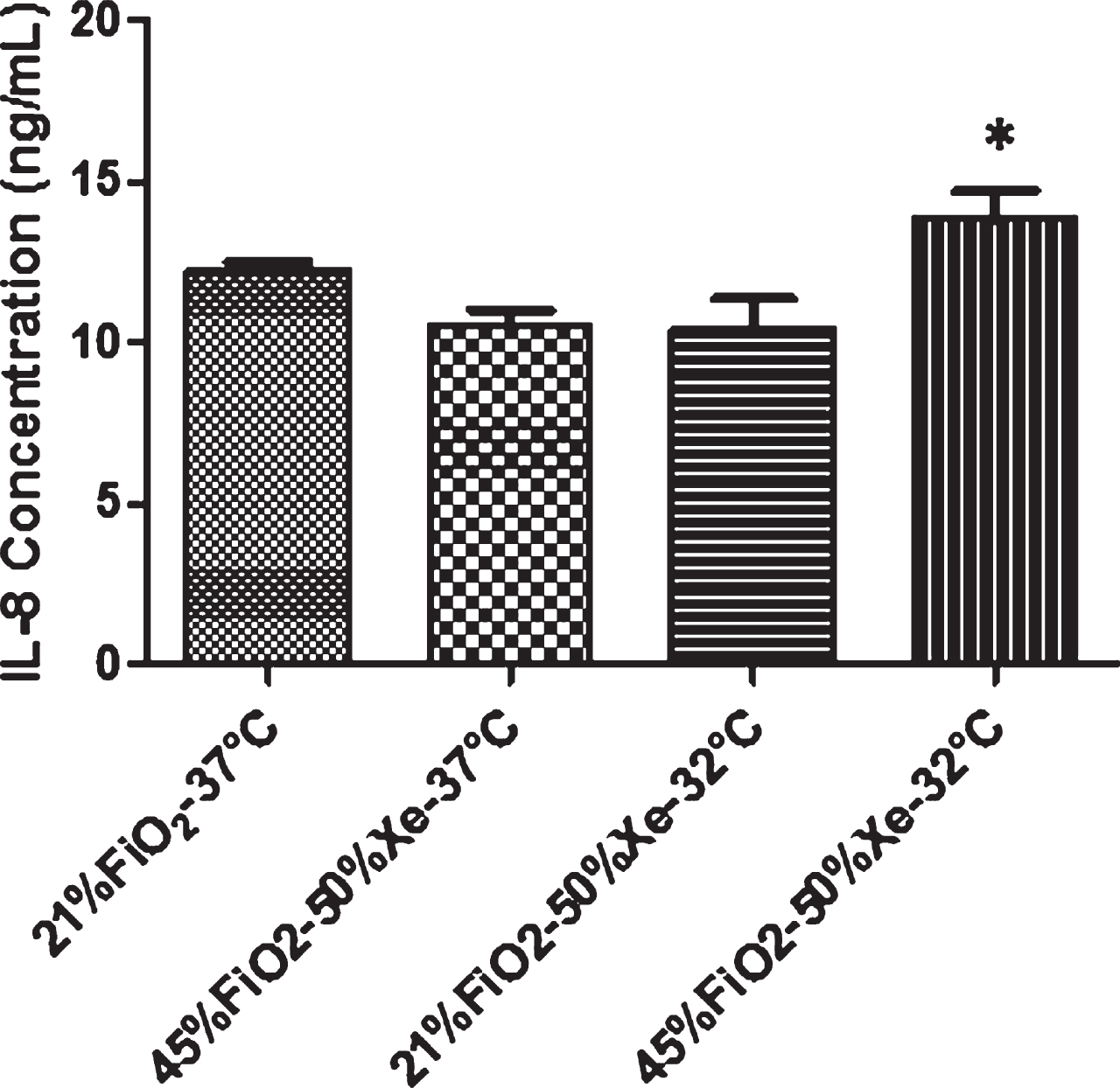
4Discussion
In this study, we evaluated the direct effects of xenon gas with mild hypothermia (32°C) in comparison with normothermia (37°C) on cultured human airway epithelial cells (Calu-3 cell line) as an in vitro model over 24 hours. We chose to use 50% xenon in this study for the following reasons: first, alveolar concentrations from 35% to 50% of xenon gas combined with hypothermia have shown neuroprotective function in hyperoxic-ischemic animal models and clinical studies [21, 31]; second, 50% xenon is achievable in this in vitro cell culture model while providing 45% oxygen and 5% CO2 to culture Calu-3 cells.
The Calu-3 cell line (American Type Culture Collection, ATCC HTB-55) is a well-differentiated and characterized cell line that is derived from human bronchial submucosal glands [41]. Calu-3 cells have been used to screen drug candidates delivered to the respiratory epithelium and to evaluate oxidant stress in respiratory disease. Other potential in vitro cell models have been employed for pulmonary research. Alveolar epithelial cells, such as A549 cells, lack many important secretory characteristics, do not form functional tight junction structures, and generate very low epithelial resistance [42]. Some studies showed that primary cells developed an inconsistent experimental phenotype and highly variable levels of TER. By contrast, Calu-3 cells formed high-resistance monolayers and expressed similar markers to primary cells, indicating that Calu-3 cells may be the most suitable model cell line for air-liquid interface experiments [43].
The TER measurement has been used to assess the permeability of tight junctions or the integrity of Calu-3 monolayer. At the 24-hour time point, for each of the experimental conditions, the TER values were normalized with the baseline TER values of the same group. The TER decreased at 24 hours in all treatment groups. Xenon with hyperoxia and hypothermia resulted in greatest decrease in TER compared with other groups. In our previous study, the Calu-3 cells exposed to 40% FiO2 oxygen did not demonstrate a decrease in TER at 24 hours [37]. In this regard, we speculate that hypothermia played an important role in the reduction of TER.
Airway epithelial integrity is maintained by paracellular tight junctions, a complex of intracellular and membrane proteins. The three major trans membrane proteins are occludin, claudins, and junction adhesion molecule proteins. Zonula occludens-1 (ZO-1), also known as tight junction protein-1, is a peripheral membrane protein, which was found on the cytoplasmic surface and endothelial cell membranes, and it is one of three major scaffold proteins concentrated at the cytoplasmic surfaces of the intercellular junctional complexes [38].
In this study, ZO-1 protein was densely localized at the boundary of the cells in the control group of Calu-3 cells. After Calu-3 cells were exposed to hyperoxia, hypothermia, and xenon for 24 hours, immunefluorescence localization of ZO-1 (XY) showed reduced density of the ZO-1 rings and incomplete ring-like staining in the hyperoxia with xenon group at 32°C compared with other groups. These results indicated that hyperoxia and hypothermia disrupt tight junction ZO-1 protein structure and result in redistribution of tight junction protein to cytoplasma.
Calu-3 cells demonstrate many of the characteristics of the bronchiolar epithelium. Previous studies have shown that, like the intact airway, the protein secretions in Calu-3 ASF are similar to those from primary airway cultures and bronchoalveolar lavage fluid [34, 35]. The level of total protein reflects the cell membrane dysfunction and injury [39, 40]. In this experiment, the treatment of xenon gas, moderate level of oxygen exposure, and mild hypothermia did not trigger the Calu-3 monolayers to secrete a high level of total protein compared with the control group over 24 hours.
IL-6, a pro-inflammatory cytokine, is produced at the site of inflammation and is an indicator of tissue damage and infection [44]. It has been described as both a pro-inflammatory and anti-inflammatory molecule [45]. IL-8, a leukocyte chemotactic-activating cytokine (chemokine), is produced by various types of cells in the presence of inflammatory stimuli such as lipopolysaccharide, IL-1, and tumor necrosis factor. It plays a causal role in the acute inflammatory cascade [46]. As shown herein, Calu-3 cells exposed to normoxia, xenon, and hypothermia secreted less IL-6 compared with the other groups. Moderate levels of oxygen with xenon and mild hypothermia triggered airway epithelial cells to secrete higher levels of IL-8 than the other groups, and xenon did not appear to be additive. Interestingly, in a study using primary human umbilical vein endothelial cells, the secretion of IL-6 increased, and IL-8, monocyte chemoattractant protein-1, and cyclooxygenase (COX)-2 protein expression decreased, after 6 hours of mild hypothermia. These findings are new mechanisms of hypothermia-induced cell protection in endothelial cell biology [47].
In conclusion, compared with control conditions, hyperoxia and hypothermia result in detrimental epithelial cell function and inflammation over a 24-hour exposure. The combination of xenon gas and hypothermia did not demonstrate a confounding effect on cell function or inflammation in an in vitro cell model.
4.1Limitations
This cell model is useful for the study of respiratory diseases, airway injury related to environmental gas concentrations (e.g., oxygen toxicity), and biophysical stresses (positive mechanical ventilation). In many cases, isolated cell preparations allow for very careful control of cell environment (e.g., temperature and gas concentrations). In addition, the air-liquid interface culture enables the direct sampling and measurement of luminal airway secretions in the absence of systemic factors. However, it should be noted that the absence of systemic inflammation, circulatory factors, and other paracrine systemic influences is a potential limitation of the isolated cell system and should be carefully considered for certain investigations. It is unfeasible to directly measure the concentrations of xenon gas because of the limitations of conventional anesthetic gas analyzers, although an ultrasonic method and laser refractometry have been used to measure xenon concentrations in breathing circuits [48]. As noted above, we indirectly measured the concentration of xenon gas in proportion to the concentration of oxygen in the inhaled gas mixtures using an oxygen analyzer (MAXO2). In this regard, we have confidence in the accuracy of xenon gas concentration measurements.
Disclosure statements
Financial Disclosure: Contents are solely the responsibility of the authors and do not necessarily represent the official views of Nemours Foundation or the NIH. The authors have no conflicts of interest to disclose.
Acknowledgments
The Nemours Foundation and NIH COBRE Grant P30 GM114736
(Thomas H. Shaffer, PI, principal investigator).
References
[1] | Perlman JM . Markers of asphyxia and neonatal brain injury. N Engl J Med. (1999) ;341: :364–5. |
[2] | Low JA . The relationship of asphyxia in the mature fetus to long-term neurologic function. Clin Obstet Gynecol. (1993) ;36: :82–90. |
[3] | Finer NN , Robertson CM , Richards RT , Pinnell LE , Peters KL . Hypoxic-ischemic encephalopathy in term neonates: perinatal factors and outcome. J Pediatr. (1981) ;98: :112–7. |
[4] | Ekert P , Perlman M , Steinlin M , Hao Y . Predicting the outcome of postasphyxial hypoxic-ischemic encephalopathy within 4 hours of birth. J Pediatr. (1997) ;131: :613–7. |
[5] | Adamson SJ , Alessandri LM , Badawi N , Burton PR , Pemberton PJ , Stanley F . Predictors of neonatal encephalopathy in full-term infants. BMJ. (1995) ;311: :598–602. |
[6] | Zhang W , Ma J , Danzeng Q , Tang Y , Lu M , Kang Y . Safety of moderate hypothermia for perinatal hypoxic-ischemic encephalopathy: a meta-analysis. Pediatr Neurol. (2017) ;74: :51–61. |
[7] | Gluckman PD , Wyatt JS , Azzopardi D , Ballard R , Edwards AD , Ferriero DM , et al. Selective head cooling with mild systemic hypothermia after neonatal encephalopathy: multicentre randomised trial. Lancet. (2005) ;365: :663–70. |
[8] | Eicher DJ , Wagner CL , Katikaneni LP , Hulsey TC , Bass WT , Kaufman DA , et al. Moderate hypothermia in neonatal encephalopathy: safety outcomes. Pediatr Neurol. (2005) ;32: :18–24. |
[9] | Sirimanne ES , Blumberg RM , Bossano D , Gunning M , Edwards AD , Gluckman PD , et al. The effect of prolonged modification of cerebral temperature on outcome after hypoxic-ischemic brain injury in the infant rat. Pediatr Res. (1996) ;39: :591–7. |
[10] | Carroll M , Beek O . Protection against hippocampal CA1 cell loss by post-ischemic hypothermia is dependent on delay of initiation and duration. Metab Brain Dis. (1992) ;7: :45–50. |
[11] | Bona E , Hagberg H , Løberg EM , Bågenholm R , Thoresen M . Protective effects of moderate hypothermia after neonatal hypoxia-ischemia: short- and long-term outcome. Pediatr Res. (1998) ;43: :738–45. |
[12] | Amess PN , Penrice J , Cady EB , Lorek A , Wylezinska M , Cooper CE , et al. Mild hypothermia after severe transient hypoxia-ischemia reduces the delayed rise in cerebral lactate in the newborn piglet. Pediatr Res. (1997) ;41: :803–8. |
[13] | Thoresen M , Bågenholm R , Løberg EM , Apricena F , Kjellmer I . Posthypoxic cooling of neonatal rats provides protection against brain injury. Arch Dis Child Fetal Neonatal Ed. (1996) ;74: :F3–9. |
[14] | Perlman JM , Wyllie J , Kattwinkel J , Atkins DL , Chameides L , Goldsmith JP , et al. Part Neonatal resuscitation: International Consensus on Cardiopulmonary Resuscitation and Emergency Cardiovascular Care Science With Treatment Recommendations. Circulation. (2010) ;122: :S516–38. |
[15] | Dingley J , King R , Hughes L , Terblanche C , Mahon S , Hepp M , et al. Exploration of xenon as a potential cardiostable sedative: a comparison with propofol after cardiac surgery. Anaesthesia. (2001) ;56: :829–35. |
[16] | Lynch C 3rd , Baum J , Tenbrinck R . Xenon anesthesia. Anesthesiology. (2000) ;92: :865–8. |
[17] | Chakkarapani E , Thoresen M , Hobbs CE , Aquilina K , Liu X , Dingley J . A closed-circuit neonatal xenon delivery system: a technical and practical neuroprotection feasibility study in newborn pigs. Anesth Analg. (2009) ;109: :451–60. |
[18] | Franks NP , Dickinson R , de Sousa SL , Hall AC , Lieb WR . How does xenon produce anaesthesia? Nature. (1998) ;396: :324. |
[19] | Robertson NJ , Tan S , Groenendaal F , van Bel F , Juul SE , Bennet L , et al. Which neuroprotective agents are ready for bench to bedside translation in the newborn infant? J Pediatr. (2012) ;160: :544–52.e4. |
[20] | Natale G , Cattano D , Abramo A , Forfori F , Fulceri F , Fornai F , et al. Morphological evidence that xenon neuroprotects against N-methyl-DL-aspartic acid-induced damage in the rat arcuate nucleus: a time-dependent study. Ann N Y Acad Sci. (2006) ;1074: :650–8. |
[21] | Wilhelm S , Ma D , Maze M , Franks NP . Effects of xenon on in vitro and in vivo models of neuronal injury. Anesthesiology. (2002) ;96: :1485–91. |
[22] | Jordan BD , Wright EL . Xenon as an anesthetic agent. AANA J. (2010) ;78: :387–92. |
[23] | Drayer BP , Gur D , Yonas H , Wolfson SK Jr , Cook EE . Abnormality of the xenon brain: Blood partition coefficient and blood flow in cerebral infarction: An in vivo assessment using transmission computed tomography. Radiology. (1980) ;135: :349–54. |
[24] | Bouma GJ , Muizelaar JP , Stringer WA , Choi SC , Fatouros P , Young HF . Ultra-early evaluation of regional cerebral blood flow in severely head-injured patients using xenon-enhanced computerized tomography. J Neurosurg. (1992) ;77: :360–8. |
[25] | Luttropp HH , Romner B , Perhag L , Eskilsson J , Fredriksen S , Werner O . Left ventricular performance and cerebral haemodynamics during xenon anaesthesia. A transoesophageal echocardiography and transcranialdopplersonography study. Anaesthesia. (1993) ;48: :1045–9. |
[26] | Hettrick DA , Pagel PS , Kersten JR , Tessmer JP , Bosnjak ZJ , Georgieff M , et al. Cardiovascular effects of xenon in isoflurane-anesthetized dogs with dilated cardiomyopathy. Anesthesiology. (1998) ;89: :1166–73. |
[27] | Thoresen M , Hobbs CE , Wood T , Chakkarapani E , Dingley J . Cooling combined with immediate or delayed xenon inhalation provides equivalent long-term neuroprotection after neonatal hypoxia-ischemia. J Cereb Blood Flow Metab. (2009) ;29: :707–14. |
[28] | Ma D , Hossain M , Chow A , Arshad M , Battson RM , Sanders RD , et al. Xenon and hypothermia combine to provide neuroprotection from neonatal asphyxia. Ann Neurol. (2005) ;58: :182–93. |
[29] | Faulkner S , Bainbridge A , Kato T , Chandrasekaran M , Kapetanakis AB , Hristova M , et al. Xenon augmented hypothermia reduces early lactate/N-acetylaspartate and cell death in perinatal asphyxia. Ann Neurol. (2011) ;70: :133–50. |
[30] | Sanders RD , Ma D , Maze M . Xenon: Elemental anaesthesia in clinical practice. Br Med Bull. (2005) ;71: :115–35. |
[31] | Hobbs C , Thoresen M , Tucker A , Aquilina K , Chakkarapani E , Dingley J . Xenon and hypothermia combine additively, offering long-term functional and histopathologic neuroprotection after neonatal hypoxia/ischemia. Stroke. (2008) ;39: :1307–13. |
[32] | Chakkarapani E , Dingley J , Liu X , Hoque N , Aquilina K , Porter H , et al. Xenon enhances hypothermic neuroprotection in asphyxiated newborn pigs. Ann Neurol. (2010) ;68: :330–41. |
[33] | Petzelt C , Blom P , Schmehl W , Muller J , Kox WJ . Xenon prevents cellular damage in differentiated PC-12 cells exposed to hypoxia. BMC Neurosci. (2004) ;5: :55. |
[34] | Zhang Y , Reenstra WW , Chidekel A . Antibacterial activity of apical surface fluid from the human airway cell line Calu- Pharmacologic alteration by corticosteroids and beta(2)-agonists. Am J Respir Cell Mol Biol. (2001) ;25: :196–202. |
[35] | Babu PBR , Chidekel A , Shaffer TH . Hyperoxia-induced changes in human airway epithelial cells: the protective effect of perflubron. Pediatr Crit Care Med. (2005) ;6: :188–94. |
[36] | Rajasekaran SA , Palmer LG , Moon SY , Peralta Soler A , Apodaca GL , Harper JF , et al. Na, K-ATPase activity is required for formation of tight junctions, desmosomes, and induction of polarity in epithelial cells. Mol Biol Cell. (2001) ;12: :3717–32. |
[37] | Zhu Y , Miller TL , Singhaus CJ , Shaffer TH , Chidekel A . Effects of oxygen concentration and exposure time on cultured human airway epithelial cells. Pediatr Crit Care Med. (2008) ;9: :224–9. |
[38] | Anderson JM , Van Itallie CM . Physiology and function of the tight junction. Cold Spring Harb Perspect Biol. (2009) ;1: :a002584. |
[39] | Chidekel A , Zhu Y , Wang J , Mosko JJ , Rodriguez E , Shaffer TH . The effects of gas humidification with high-flow nasal cannula on cultured human airway epithelial cells. Pulm Med. (2012) ;2012: :380686. |
[40] | Zhu Y , Chidekel A , Shaffer TH . Cultured human airway epithelial cells (Calu-3): a model of human respiratory function, structure, and inflammatory responses. Crit Care Res Pract. (2010) ;2010: :394578. |
[41] | Shen BQ , Finkbeiner WE , Wine JJ , Mrsny RJ , Widdicombe JH . Calu- A human airway epithelial cell line that shows cAMP-dependent Cl- secretion. Am J Physiol. (1994) ;266: :L493–501. |
[42] | Stewart CE , Torr EE , Mohd Jamili NH , Bosquillon C , Sayers I . Evaluation of differentiated human bronchial epithelial cell culture systems for asthma research. J Allergy (Cairo). (2012) ;2012: :943982. |
[43] | Winton HL , Wan H , Cannell MB , Gruenert DC , Thompson PJ , Garrod DR , et al. Cell lines of pulmonary and non-pulmonary origin as tools to study the effects of house dust mite proteinases on the regulation of epithelial permeability. Clin Exp Allergy. (1998) ;28: :1273–85. |
[44] | Tanaka T , Narazaki M , Kishimoto T . IL-6 in inflammation, immunity, and disease. Cold Spring Harb Perspect Biol. (2014) ;6: :a016295. |
[45] | Scheller J , Chalaris A , Schmidt-Arras D , Rose-John S . The pro- and anti-inflammatory properties of the cytokine interleukin-6. Biochim Biophys Acta. (2011) ;1813: :878–88. |
[46] | Harada A , Sekido N , Akahoshi T , Wada T , Mukaida N , Matsushima K . Essential involvement of interleukin-8 (IL-8) in acute inflammation. J Leukoc Biol. (1994) ;56: :559–64. |
[47] | Diestel A , Roessler J , Berger F , Schmitt KRL . Hypothermia downregulates inflammation but enhances IL-6 secretion by stimulated endothelial cells. Cryobiology. (2008) ;57: :216–22. |
[48] | King R , Bretland M , Wilkes A , Dingley J . Xenon measurement in breathing systems: A comparison of ultrasonic and thermal conductivity methods. Anaesthesia. (2005) ;60: :1226–30. |