Therapeutic Potential of Microbiota Modulation in Alzheimer’s Disease: A Review of Preclinical Studies
Abstract
Alzheimer’s disease (AD) is the most common neurodegenerative disease, yet it currently lacks effective treatment due to its complex etiology. The pathological changes in AD have been linked to the neurotoxic immune responses following aggregation of Aβ and phosphorylated tau. The gut microbiota (GM) is increasingly studied for modulating neuroinflammation in neurodegenerative diseases and in vivo studies emerge for AD. This critical review selected 7 empirical preclinical studies from 2019 onwards assessing therapy approaches targeting GM modulating microglia neuroinflammation in AD mouse models. Results from probiotics, fecal microbiota transplantation, and drugs were compared and contrasted, including for cognition, neuroinflammation, and toxic aggregation of proteins. Studies consistently reported significant amelioration or prevention of cognitive deficits, decrease in microglial activation, and lower levels of pro-inflammatory cytokines, compared to AD mouse models. However, there were differences across papers for the brain regions affected, and changes in astrocytes were inconsistent. Aβ plaques deposition significantly decreased in all papers, apart from Byur dMar Nyer lNga Ril Bu (BdNlRB) treatment. Tau phosphorylation significantly declined in 5 studies. Effects in microbial diversity following treatment varied across studies. Findings are encouraging regarding the efficacy of study but information on the effect size is limited. Potentially, GM reverses GM derived abnormalities, decreasing neuroinflammation, which reduces AD toxic aggregations of proteins in the brain, resulting in cognitive improvements. Results support the hypothesis of AD being a multifactorial disease and the potential synergies through multi-target approaches. The use of AD mice models limits conclusions around effectiveness, as human translation is challenging.
INTRODUCTION
Alzheimer’s disease (AD) is a neurodegenerative disease characterized by the loss of neuronal structure and function, affecting memory formation and cognition [1]. The social and economic burden of AD is growing, with devastating effects for patients and a heavy impact on their caregivers [2]. It is hypothesized that AD originates from the deposition of amyloid-β (Aβ) peptide that form plaques and neurofibrillary tangles associated with tau protein [3]. However, late-stage trials centered on targeting Aβ or tau protein have been continuously failing and treatment options lack [4].
In vitro and in vivo studies have shown that many proteinopathies like AD stimulate neuroimmune responses, which have a key role in the onset and progression of the disease [5]. Microglia are the primary immune cells of the central nervous system (CNS), with proinflammatory and neuroprotective roles; they constitute 5–10% of total brain cells [6]. In AD, chronic low-level activation of microglial cells may lead to neurotoxicity and pathological changes [7]. Therefore, researchers highlight the need to identify novel therapeutic targets and develop treatments that modulate immune and inflammatory processes in the brain [8, 9].
Emergent evidence emphasizes the potential role the gut microbiota (GM) may have in modulating neuroimmune functions, beyond the gastrointestinal tract through the brain-gut axis. Preclinical data examining GM-brain interactions suggest that the gut microbiome is key in regulating microglial maturation and activation in homeostatic conditions via short-chain fatty acids (SCFAs) [10, 11]. In addition, reduced microbiota complexity has shown to change microglia properties and result in defective microglia [11]. Gut dysbiosis, defined as a microbial compositional imbalance exerting a pathobiological effect, and GM-host interactions appear to hold a key role in neurodegeneration [12]. Several findings highlight that the accumulation of misfolded proteins, axonal damage, and neuronal demyelination linked to gut dysbiosis facilitate the pathogenesis of neurodegenerative disorders like Parkinson’s disease, multiple sclerosis, amyotrophic lateral sclerosis, and AD [13]. Primary research on alternative therapeutic approaches targeting gut dysbiosis are growing as they are believed to hold promise for altering disease pathogenesis through varying mechanisms [10]. Nevertheless, critical reviews of the latest therapeutic findings for treating AD are missing.
The aim of this paper is to critically review the latest papers examining GM modulation of microglia in AD in order to evaluate the quality of current data, including its potential contradictions or gaps. This is important to establish a basis for the future research needs in the treatment of AD. The first half aimed to outline and review the selected literature whilst the second half looked to critically review the methodologies and analysis of the selected papers, with a focus on the study designs and measurements for the GM, AD biomarkers, neuroinflammation, and cognition.
The studies reviewed here were found through a PubMed search as well as hand searching references from other reviews (see the Supplementary Material for the keywords used and search details). Inclusion criteria comprised a publication date from 2019, each study needed to come from a different research group and studies needed to assess a treatment approach acting on microbiota modulation of microglia in AD.
LITERATURE REVIEW
Ongoing research provides early evidence that food-based therapy, probiotic supplementation, prebiotics, fecal microbiota transfer (FMT), or drug treatments can improve several brain diseases by regulating the GM [14]. For AD, the approach is based on the evidence that patients display reduced microbial diversity, as indicated by fecal analysis compared to controls (see Fig. 1) [15]. This is linked to the gut barrier breakdown, further increase of proinflammatory cytokines (through the release of amyloids and liposaccharides from bacteria), and bacteria-derived products in circulation [16]. The systemic inflammation leads to blood-brain barrier (BBB) impairment and neuroinflammation through microglia activation [17]. Activation happens because microglia detect the impairment via soluble molecules that carry humoral information [18]. Over the last five years, the number of publications exploring this hypothesis has grown exponentially with a strong interest in gut-brain axis research [19]. However, it remains unclear whether Aβ-related alteration of GM is causal to neuroinflammation or/and vice versa [14].
Fig. 1
Microbiota-gut-brain axis involvement in AD pathogenesis. The gut microbiota disturbances (changes in bacteria diversity) lead to an increase in gut barrier permeability that may cause release of molecules in systemic circulation (including proinflammatory cytokines) and an increase in the BBB permeability. This may in turn activate microglia (changing their morphology) and lead to an accumulation of Aβ. The neuroinflammation and neurodegeneration that follow are characteristic of AD. SCFA, short chain fatty acids; IL, interleukin; TNF, tumor necrosis factor; BBB, blood-brain barrier.
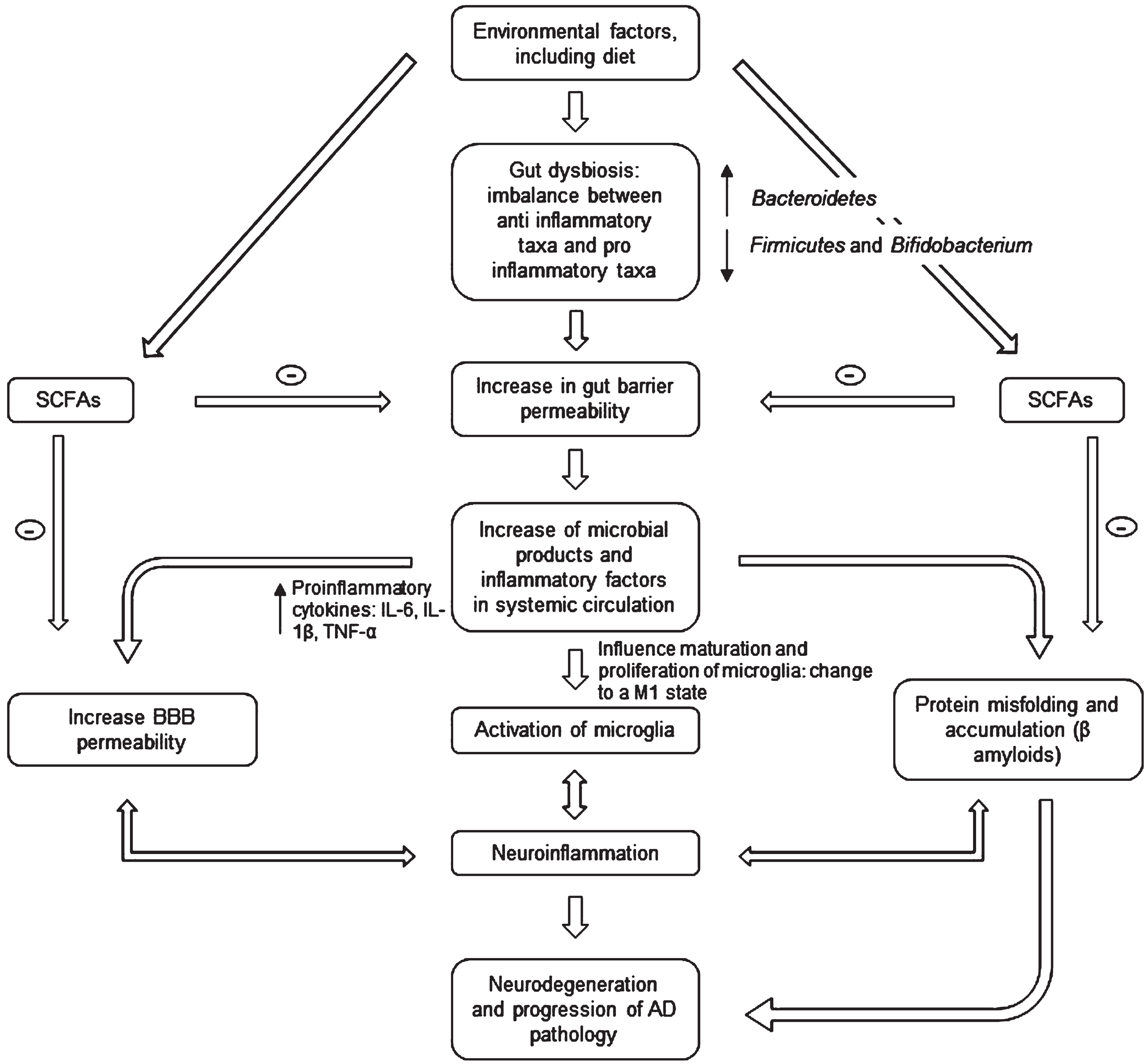
A selection of the latest AD preclinical research focused microbiota-linked therapies is discussed (see summary of included studies in Table 1); this is not claiming to be a complete review of all studies conducted.
Table 1
Summary of the primary studies included in the literature review
Treatment (length) | Type | Model(s) | Stage of disease | Results | Year | Authors |
Clostridium butyricum | Probiotic, 4 weeks, intragastrically | APP/PS1 and WT mice | 6 months old | Prevention of cognitive impairment, Aβ deposits, microglia activation, and production of TNF-α and IL-1β in the brain. | 2019 | Sun et al. [20] |
Sodium oligomannate | Oligosaccharide anti-AD drug, 3 months, orally | 5XFAD, APP/PS1 and WT mice | 9 months old | GM reconditioned, decreased the concentration of phenylalanine and isoleucine, reduced Th1 neuroinflammation, Aβ, tau, cognitive improvement | 2019 | Wang et al. [21] |
Fecal transplantation | Fecal microbiota transfer, 4 months, orally | ADLPAPT and WT mice | 2 months old (shows amyloid plaques, neurofibrillary tangles, reactive gliosis in brains, memory deficits) | Mitigation of Aβ plaque (and both soluble and insoluble forms in the cortex) and tau tangle formations, memory deficit, reactive gliosis, change in colonic gene expression | 2020 | Kim et al. [22] |
Agathobaculum butyriciproducens | Probiotic, 10 weeks, orally | APP/PS1, lipopolysaccharide (LPS) and B6 mice | 5 months old (early onset AD) | Cognitive improvement, increased IGF-1 gene expression and upregulated NGF mRNA, reduced Aβ, decreased microglia activation (no significant for astrocytes), reduced expression of IL-1β mRNA in cortex, decreased C1QB mRNA expression | 2021 | Go et al. [23] |
Bifidobacterium breve | Probiotic, 4 months, orally | AppNL–G–F and vehicle mice | 3 months old (before memory impairment, normally exhibited at 6 months old) | Prevented cognitive impairment and attenuated microgliosis, increased ADAM10 level and Aβ in hippocampus, reduced IL-1β and IL-6 and increased TGF-β1, no marked change on GM | 2022 | Abdelhamid et al. [24] |
BdNlRB | Tibetan medicine, containing 25 kinds of herbs and minerals, 8 weeks, orally | Aβ1–40 protein-induced, APP/PS1 and WT mice | 6 months old | Improved learning ability (not significantly for memory ability), alteration of protein expression in hippocampus, P-tau decrease, no Aβ decrease, increase in number of microglia, positive regulation of intestinal flora | 2022 | Tsering et al. [25] |
Combination of Anti-Aβ Antibody NP106 and Curcumin Analog TML-6 | Combination treatment, 17 weeks, orally (ad libitum) | APP/PS1 and WT littermates | 13 weeks old (early / prodromal AD) | attenuated brain Aβ and insoluble Aβ (not for soluble forms), improved the nesting behavioral deficit (superior results in combination therapy than monotherapy), enhanced microglial Aβ phagocytosis, reduced IL-6 and TNFα cytokines, normalized GM and more similar bacterial communities to WT | 2022 | Su et al. [26] |
All the studies reviewed here are based on the potential to modify the microbiota and neuroimmune response as a way to decrease AD symptoms and biomarkers. They propose diverse innovative preventative and therapeutic options against AD. For this, they assessed different parameters across the brain-gut axis:
In vivo studies driven by the common hypothesis of butyrate
Several probiotic studies for AD reported butyrate as a main actor involved in the mechanism of action. Butyrate is a SCFA produced by specific microbes in the gut by anaerobic fermentation of dietary fiber; as a SCFA, it is a functional component of microbe-to-host signaling and crosstalk [27]. Physiological functions like energy homeostasis, immune system regulation, brain function, and obesity depend on butyrate, which acts as an energy source and potent regulator [28]. Clostridium butyricum (CB) is a butyrate-producing probiotic from the GM that has been tested in vivo for treatment of AD. The hypothesis from the study of Sun et al. [20] is based on previous evidence that butyrate modulates abnormal GM, suggesting that after being produced in the colon, it can bypass portal circulation and reach the brain. Butyrate enhances the anti-inflammatory activity of peroxisome proliferator-activated receptor γ and exerts neuroprotective effects [26]. In addition, authors found decreased butyrate levels in APP/PS1 mice compared to wild-type (WT) but increased after treatment. Agathobaculum butyriciproducens (SR79) is a strict anaerobic bacterium, which is also butyric acid-producing. The research conducted by Go et al. [23] assessing SR79 in AD was based on the same hypothesis. Other SCFAs identified as signaling molecules include acetate and propionate, which can also engage inhibitory mechanisms reducing the secretion of cytokines by immune cells, therefore regulating microglia function [29]. Propionate, which possesses similar plasma concentration and FFAR3 receptor affinity to butyrate, has previously shown to have protective effects upon BBB endothelial cells in vitro. Probiotic based on Bifidobacterium breve (MCC1274) has also been studied as this is an anti-inflammatory bacteria secreting gamma-aminobutyric acid which can regulate brain functions. Findings that associate AD with a low abundance of MCC1274 support this hypothesis [23].
Therapeutic-induced reversion of abnormal GM composition
Research in the area has still not reached a consensus over the GM perturbations linked to AD and the GM changes induced by treatments. Certain studies show that decrease in fecal microbiota diversity is associated with AD patients or with different GM composition than that of healthy mice [22]. Indeed, human and mice studies investigating FMT showed community-level alterations in AD, which induces chronic inflammation [22]. In the experiment with ADLPAPT mice models, FMT from healthy WT mice normalized gut homeostasis.
Some have not identified significant differences, for instance between APP/PS1 mice and CB-treated mice, using the Shannon and Simpson index for α-diversity [20]. Also, Abdelhamid et al. [24] did not find a marked change in the GM following probiotic treatment, which could be explained by the involvement of structural components in the effects. Sun et al. [20] suggest that greater microbe richness does not imply a healthy GM but instead could be attributed to varied harmful bacteria. However, treatment helps in having bacterial communities more akin to the WT mice [26]. After treatment, GM composition showed a significant increase of Deferribacteres, Alloprevotella and S24-7 and decrease of Helicobacteraceae [20]. This shows a reversion of abnormal GM composition, which is confirmed by the study of Su et al. using 16S rDNA Sequencing, where decrease of Alloprevotella and Anaeroplasma is reversed and higher levels Butyricicoccus of the family Ruminococcaceae are observed but it mentions also other bacteria lowered by the treatment, Dubosiella, Parabacteroides, Bacteroides, and Rikenellaceae RC9 [26].
While the majority of studies included an assessment of the gut microbiota, as a parameter of treatment mechanisms and effect, one of them did not [23]. Nevertheless, we observe a common modification of the GM with different conclusions around the specific bacterial variations as it is likely that different therapeutic approaches modify in different ways the microbiota. These findings support the effectiveness of therapies in reversing varying GM abnormalities in AD.
Systemic and CNS inflammation
Several of the reviewed papers highlighted the contributing effects of systemic inflammation in neurodegeneration [20, 23]. It appears that alterations in GM composition led to increased intestinal permeability before chronic systematic inflammation with circulating Ly6C+inflammatory monocytes and could accelerate AD via signaling molecules produced to the brain [22]. FMT from controls allows the decrease of gut permeability compared to FMT from AD mice models, changing colonic gene expression and peripheral immune cell population [22]. This potentially happens by regulating concentrations of phenylalanine and isoleucine in amino-acid-related metabolic pathways and enzymes that could be taken by immune cells through specific transporters, driving immune cell differentiation and proliferation [21]. Sodium oligomannate (GV-971) treatment seems to harness the infiltration of peripheral Th1 cells into the brain via blood circulation. The GV-971 drug is a mixture of acidic linear oligosaccharides and is tested for its effects against AD because oligosaccharides are the primary nutrient source for bacteria, with evidence showing their ability to modulate bacteria [21]. This brings new perspectives in approaching AD as a systemic disease, with the opportunity to target both its peripheral and central manifestations [31, 32]. However, mechanisms and putative effects need further investigation.
Current evidence also suggests an anti-inflammatory function of probiotics by decreasing microglia activation whereas no significant changes are found for astrocytes [23, 24]. This explains the reduction in mRNA expression of IL-1β, IL-6, or TNF-α cytokines in the brain (pro-inflammatory) and increases in TGF-β1 (anti-inflammatory) mRNA expression levels [20, 23, 24].
Other papers observe an increase in microglia, suggesting that microglia may rejuvenate and change their morphology from a M1 proinflammatory form into their M2 neuroprotective form that enhances Aβ phagocytosis [21, 25]. This confirms the hypothesis of the comprehensive study by Wang et al. [21], that is based on the evidence that the GM is likely involved in regulated microglia activation and neuroinflammation in AD.
Therefore, current evidence supports a beneficial effect of such therapies on neuroinflammation observed in AD. However, papers reviewed did not highlight sex-dependent changes, which contrasts with other papers, such as the one from Dodiya et al. that identifies sex-dependent alterations in microglia morphology and variations of brain Aβ plaques after perturbation of the gut microbiome, only in male mice compared with female mice [21, 33].
AD established biomarkers: Amyloid deposition and tau phosphorylation
A general conclusion of the studies is the significant decrease in amyloid plaque deposition, Aβ40, Aβ42, and insoluble Aβ levels [20, 24]. The reduction observed may occur via the increase of ADAM10 protein of AβPP, which cause the inhibition of Aβ production [24]. However, there are differences across studies on whether significant changes apply to the cortex and/or hippocampus, on the level of decrease in soluble forms of Aβ or tau phosphorylation, when measured using immunohistochemical assays with confocal imaging [24, 26]. The reasons for these differences are unclear, according to Abdelhamid et al. [24], probiotics may activate specific brain regions through the vagus afferent nerve. However, depending on the dose and the pharmacological approach, the treatment can cause more damage than improvement [25, 26]. Significant increase in Aβ plaques and gastrointestinal burden have been observed when testing high doses of a classic Tibetan medicine called Byur dMar Nyer lNga Ril Bu (BdNlRB). BdNlRB contains 25 kinds of herbs and minerals and is traditionally used for treating “white vein disease”, referring to CNS disease [25]. Despite this, overall findings support the significance of AD biomarkers improvements with low or moderate doses.
Neurodegeneration and neurogenesis
In order to achieve cognitive improvements, neuronal functioning is essential and can be measured [34]. Neuroprotective properties are observed after probiotic treatment by the increase of synaptic protein levels and significant reduction of degenerative neurons, indicated by FJC staining [20, 24]. Neurotrophic factor expression (such as IGF-1, AKT, GSK3β), which is critical for neural plasticity and neurogenesis, was upregulated by chronic SR79 treatment when performing real-time quantitative polymerase chain reaction (RT-qPCR) [23]. However, it has also been identified that cell proliferation in the hippocampus does not significantly differ after probiotic treatment, which could suggest an improvement of cognition via increased hippocampal synaptic density [24]. This can be explained by the inhibition of neuronal apoptosis and repair of neuronal function by amino acid synthesis and neurotransmitter metabolism, which depends on protein expression alterations [25]. It aligns with the beneficial role of IGF-1 in neuronal survival and synaptic plasticity, and its link with cognitive decline when disrupted [23]. Lack of neuronal and synaptic analysis in all individual studies challenges the generalization of results.
Prevention and improvement of cognitive impairment
Cognition assessment is key to measure the efficacy of an AD treatment. Cognition improvement and prevention of deficits is observed in mice after treatment with probiotics starting before they exhibit deficits [20, 24]. For instance, oral supplementation with probiotic MCC1274 significantly prevented memory impairment, using Novel Object Recognition (NOR) test, in 7-month-old AppNL-G-F mice that would normally exhibit memory impairments from 6 months of age. Treatment with probiotic CB allowed 6-month-old mice to distinguish familiar from novel objects and showed a notable reduction in the escape latency, compared to APP/PS1 mice.
The amelioration of impairment is observed across mice of different ages representing varying stages of AD [21]. Also, results are significant and consistent across different mice models of AD [23]. However, BdNlRB did not improve memory ability in APP/S1 mice despite significant learning improvements [25]. It is possible that only some components of this multi-target approach could enter the brain. The study designed by Su et al. [26] also follows a synergistic approach by arguing it is better suited for AD given its multifactorial etiology. It uses the promise of passive immunotherapy using an anti-Aβ antibody NP106 for slowing down Aβ accumulation (data from clinical trials). Combined to it, curcumin analogue TML-6 allows neuroactive effects of SCFAs on microglial function for decreased neuroinflammation, through the alteration of bacterial communities (including Butyricicoccus). In this case, the treatment managed to reverse symptoms more than monotherapy, being statistically comparable to controls [26]. These results are in line with a study about immunotherapy for the treatment of AD targeting tau that has recently showed its ability to modulate the GM in an AD mouse model [35]. Authors speculate that the GM modulation may underlie the treatment efficacy and encourage the further exploration of multitarget and combinatorial therapies [35]. Lastly, FMT improved short-term and long-term memory thanks to continuous exposure to healthy microbiota [22].
Together, these studies bring confirmation that approaches modulating the GM are effective in improving cognitive functioning. However, for pharmacological and combination approaches, penetrating the BBB is challenging which can result in low drug concentrations in the brain and lower efficacy [26].
REVIEW OF STUDY METHODOLOGIES
Animal models and study design
Up to now, the majority of data regarding the GM-microglia interactions in AD treatments is based on preclinical data with mice, whereas only a few are clinical studies [12, 36]. In this review, all papers used AD mouse models to measure the effect of their specific interventions on AD pathophysiology and symptomatology (see Table 1). Numerous genetically modified mice are available for research and there is an advanced knowledge on these models that present anatomical and physiological similarities [37]. However, they also present some limitations due to specific morphological differences with humans, such as larger relative length of the small intestine, different dietary requirements, and higher specific metabolic rate [38]. On top of this, it can be challenging to be aware of any variation in mouse diet, for example as many mouse chows contain varying amounts of soy influencing disease outcomes via phytoestrogens [39]. It is also important to consider that laboratory mice still have notably different gut microbiomes from their wild relatives [38].
APP/PS1 models of AD were used in 5 papers out of the 7 reviewed (see Table 1), which facilitates the comparison between studies. The CNS neurons of these mice express chimeric mouse/human amyloid precursor protein and a mutant human presenilin 1. Both mutations are associated with early-onset AD in this chronic and long-term model [40]. It is characterized by significantly different species and abundance of intestinal flora compared to normal mice, as well as the progressive development of Aβ plaques and cognitive deficits [25]. However, the age at which mice started treatment differed a lot within studies, going from 13 weeks old to 9 months old, reflecting the modelling of different stages of AD. Such heterogeneity could represent confounding factors if not considered when comparing publications’ results, for example by overlooking stage-specific efficacy levels due to age-related decline in learning capacity [41]. Also, it has been argued that APP transgenic mouse models are better suited to test prevention strategies of AD instead of treatment itself, whereas AD is often detected late when symptoms are already present [42]. Therefore, predictive validity in these models is limited, with studies having failed to translate results into trials for individuals with clinical disease [43].
Obtaining results from multiple lines of mice, as per the study by Go et al. [23], using both APP/PS1 and lipopolysaccharide (LPS) models with controls, is recommended by Zahs et al. [42] to strengthen validity and reliability before moving to clinical trials. Such approaches help in identifying whether differences in results can be attributed to differences in models. For instance, Tsering et al. [25] did observe differences in significances of learning ability improvements for APP/PS1 and Aβ1-40 mouse models.
The study design selected was consistent across papers. Experimental research designs are appropriate to measure the effect of a therapy in AD models and investigate causal relationships [44]. In all papers, the experiments were conducted with AD controls and/or WT mice. The dependent variables included GM diversity, accuracy in cognitive tasks, levels of neuroinflammation and biomarkers for AD, while the independent variable was the treatment approach (probiotics, FMT, or pharmacotherapies). Table 2 summarizes the methods used for measuring the variables. Controlled settings present advantages through their minimization of confounding factors and allowed researchers to manipulate specific parameters; however, external validity is limited [45]. In real-life settings, existing confounding factors may include concomitant diseases, choice of diet, and a history of long-term drug intake [12]. Linked to this, these mouse study designs do not consider human-specific environmental factors (for example, lifestyle, and behaviors) as well as geography, given that depending on locations, specific bacterial taxa are dominant in normal conditions [36]. In other words, while efficacy can be assessed, the measurement of effectiveness presents limitations. When possible, supporting animal data with analysis of human fecal or blood samples increases the translational impact of research, which was the approach of Wang et al. [21].
Table 2
Table summarizing treatments and measures of the primary studies reviewed
Treatment (size; sex; dose) | GM analysis | Neuroinflammation (cytokines, microglia &astrocytes) | AD biomarkers (Aβ; tau) &Neurodegeneration | Cognition |
Clostridium butyricum n=10; n = 10 WT: n unknown sex unknown 1×109 cfu mL - 1/200μL/day | Fecal sample DNA extraction and amplification with 16S rRNA using Illumina MiSeq sequencing, PCA and LDA analysis | Levels of IL-1β and TNF-α (proinflammatory cytokines) were detected using ELISA.
Microglia: immunofluorescence and western blot analysis with antibodies (CD11b, COX-2, p-NF-κB p65) | ELISA for Aβ42 Histology with Staining using Fluoro-Jade C for degenerating neurons and Congo red for amyloidosis. | MWM NOR |
Sodium oligomannate n = 17–20 male and female 50 and 100 mg/kg/day | Fecal sample DNA extraction, PCR amplification and sequencing on Illumina MiSeq sequencing | Immunohistochemistry: Brain sections were stained for activated microglia using rabbit anti-Iba1 antibody | Immunohistochemistry: Staining for Aβ depositions and tau phosphorylation via antibodies (mouse anti-Aβ42 antibody, mouse anti-PHF, Thr231 antibody) | MWM Y-maze |
Fecal transplantation n = 13, n = 16, n = 16 sex unknown dose: not applicable | Fecal sample DNA extraction, 16S rRNA sequencing analysis using Illumina MiSeq sequencing | Immunostaining for gliosis with representative confocal images of microgliosis (ionized calcium-binding adaptor molecule; Iba1) and/or astrogliosis (glial fibrillar acidic protein; GFAP) staining in the frontal cortex | Immunohistochemistry with representative confocal images for Aβ and tau in hippocampus and frontal cortex For tau: AT180 (represent early event) and AT8 (represent middle or late stage) antibodies Aβ plaque (Biotin-4G8) staining ELISA analysis for Aβ levels in cerebral cortex | Y-maze Contextual fear conditioning, open field |
Agathobaculum butyriciproducens 3 groups of n = 8 male mice 2×108cfu/day | Not Applicable | qRT-PCR analysis of mRNA expression levels of genes IL-1β and TNF-α, IL-6, iNOS, IL-10, IL-12 (among others) to measure cytokine levels. Immunohistochemical assays incubating sections with primary antibodies (Iba1, GFAP) in cerebral cortex and hippocampus | Western blot analysis using the primary antibodies of amyloid precursor proteins (APP, pAPP, ADAM10, BACE1, Aph1a, PEN2, IDE, CD10) to measure APP processing and degrading enzyme. Measurement of neurogenesis with the expression of IGF-1 and phosphorylation of AKT and GSK3β (neurotrophic factors): p-Ser473-AKT, AKT, phospho-Ser9-GSK3β, GSK3β, β-actin) Immunohistochemistry for Aβ deposition indicated by Bam-10 immunoreactivity in parietal cortex and hippocampus | NOR Y-maze |
Bifidobacterium breve 2 groups of n = 26 sex unknown 1×109cfu/5.56 mg/200μl saline (5 times/week) | Fecal sample DNA extraction, 16S rRNA sequencing, PCoA analysis using QIIME2 software | Immunofluorescence staining for Iba1 and GFAP (using anti-82E1 and anti-Iba1 or anti-GFAP antibodies). Western blotting with primary antibodies (GFAP, Iba1) Quantitative real-time PCR (qRT-PCR) analysis of genes IL-1β and TNF-α, IL-6 to measure cytokine levels. | Immunofluorescence staining for BrdU (neurogenesis)Western blotting with incubation with primary antibodies (ADAM10, APP, BACE1, sAβPPβ, 6E10, PSD95, SYT,
HIF-1α, PKC, ERK, phospho-tau, actin, total tau) qRT-PCR for ADAM10, GAPDH. Aβ using ELISA from hippocampal and cortical tissues (for both Aβ40 and Aβ42). Immunohistochemistry for Aβ, incubating sagittal brain sections with anti-82E1 antibody that recognizes both Aβ40 and Aβ42. Images using a microscope. Quantification performed by software | NOR |
BdNlRB 2 groups of n = 60 and a control group n = 15 male mice 100, 200 and 400 mg/kg *weight | Fecal sample DNA extraction, 16S rRNA sequencing analysis using Illumina MiSeq sequencing | Immunofluorescent staining used for staining Iba1 for expression of microglia Proteomics used to compare protein expression from hippocampus across groups (observations on phosphorylated tau, microglia, phagocytosis, among others) | Expression of Aβ protein detected by immunofluorescent staining with Aβ1–42 of hippocampal samples Immunofluorescent staining used for staining p-tau (s396) for expression of phosphorylation of tau protein | MWM |
Combination of Anti-Aβ Antibody NP106 and Curcumin Analog TML-6 n = 20 sex unknown TML-6 at 100 mg/kg/day and NP106 at 3 mg/kg | Fecal sample DNA extraction from colons of mice, amplification and 16S rRNA sequencing analysis using Illumina MiSeq sequencing | Immunoreactivity of Iba1 for colocalization of microglia Morphology analysis of microglia with MetaMorph imaging software ELISA to measure proinflammatory cytokines (IL-1β, IL-6 and TNFα) | Aβ deposition as measured by Amylo-Glo staining reagent Confocal microscopy for examination of Aβ deposits and quantification in cortical and hippocampal regions with MetaMorph ELISA to measure Aβ, soluble and insoluble forms | Nesting |
Regarding the delivery of treatments, oral gavage was the most common approach in this review; however, other studies administer probiotics via drinking water [46]. Differences in routes can impact findings, as the stress induced by oral gavage can confound results [47]. The treatment duration was heterogeneous across papers, going from 4 weeks to 17 weeks, on a daily basis for all (see Table 1). Differences are also observed regarding doses, for example with 1×109 CFU or 1×108 CFU for probiotics, while the recommended minimum number of viable probiotic cells is 1×109 CFU per day [48]. Across the different types of therapies, comparing doses and dosages is challenging. Determining the optimal dose also remains difficult as efficacy is influenced by various factors, including the type of probiotics or pharmacotherapy used and the route of administration [49]. Only the studies assessing BdNlRB and sodium oligomannate compared different doses, which also helps in the identification of side effects.
Measurement techniques
Analysis of GM was performed in a standardized manner, with DNA extraction from fecal samples before amplification and 16S rRNA sequencing analysis using Illumina MiSeq sequencing in most of the cases (Table 2). A known challenge in such sequencing is the use of potentially insufficient sequencing depth to analyze microbial composition, which can lead to different proportions of groups [50]. According to Shin et al. [50], ratifying to an even depth of sequences for all sample types allows to avoid the generation of bias. In addition, analyzing different types of samples is valuable to eliminate confounding variables linked to methods and increase validity [26]. For instance, Wang et al. [21] performed a characterization of the fecal metabolome using a metabolomics non-targeted technique with fecal samples, matching the metabolites with databases, which helps limit bias and provides further level of detail [51]. Pathway enrichment analysis of metabolites revealed changes in amino acidic-related metabolic pathways and enzymes. Following this, examination of the concentrations of selected amino acids was performed in both fecal and blood samples [21].
In order to measure established biomarkers of AD, amyloid deposition and tau aggregates, the majority of studies used immunohistochemistry, which is an established method thanks to its validity and reliability [52]. To do so, researchers rapidly performed freezing or immersion of tissue in fixative to minimize tissue degradation [25]. Brain sections were stained to detect Aβ deposits and tau with the help of specific antibodies. However, different antibodies were used across papers for immunoreactivity. Only Sun et al. [20] used the classical histochemical Congo red to stain for amyloid, while the other papers used mouse anti-Aβ42, anti-82E1, or Biotin-4G8 antibodies. To measure amyloidosis, double staining with Congo red and immunohistochemistry can help in increasing specificity and avoiding false positives [53]. Four papers used immunohistochemistry in combination with ELISA analysis for Aβ levels (see Table 2). These complementary approaches showed consistent findings in these studies, which supports validity [24]. Following this, confocal microscopy allowed the visualization of results and software were used to quantify Aβ deposits, such as MetaMorph [26]. Nevertheless, not all papers collected their brain samples from the same regions for the procedures mentioned; some looked at the hippocampus and cerebral cortex while others only at the hippocampus, which limits the scope of their analysis [25].
This same procedure was applied to identify neuroinflammation with microglia. In the majority of studies reviewed, immunostaining was conducted using the antibodies of ionized calcium-binding adaptor molecule (Iba1) for microgliosis and glial fibrillar acidic protein (GFAP) for astrogliosis [22]. A limitation is the lack of specific microglia phenotype markers and morphological analysis for all papers, which will be needed to test the hypothesis of a transition from M1 to M2 state induced by GM treatments [24]. Two papers contrasted immunofluorescence results with western blotting to measure activated microglia via antibodies for Iba1 or both CD11b and COX-2 [20, 24]. To complete the analysis of neuroinflammation, researchers measured mRNA expression-levels of cytokines by qRT-PCR [23]. For instance, Go et al. [23] measured IL-1β, TNF-α, IL-6, IL-12 proinflammatory cytokines and IL-10 anti-inflammatory cytokines. qRT-PCR has been validated for reliably and quantitatively assessing in vitro immune-related gene profiles, in particular for cytokines [54]. Synaptic plasticity and neurogenesis were also assessed in four of the studies by quantifying synaptic proteins or neurotrophic factor expression via qRT-PCR, among others. However, due to word limits it will not be covered here in depth.
To evaluate prevention and improvement of memory deficits, the methods varied but all are recognized tests for memory measurement in mice. The Morris Water Maze (MWM) test was used to measure spatial learning and memory abilities through space exploration and latency time [20, 21]. MWM has proven to be a robust and reliable test that is strongly correlated with hippocampal synaptic plasticity [55]. However, researchers identified that, to reliably observe differences, a higher number of mice are necessary than those included in papers here, with 35–160 mice/group [42]. This highlights the need to define alternative tests that require smaller, more practical numbers of mice. Another test used is the novel object recognition (NOR) test for evaluating short-term memory function via exploration, identified as sniffing and touching [24]. Go et al. [23] and Abdelhamid et al. [24] scored length and times of touching by blinding an observer to the treatment; this practice decreases the risk for bias in data collection. Sun et al. [20] and Wang et al. [21] randomly allocated mice into different groups before treatment and for behaviour tests. However, blinding and randomization in animal study design is still lacking or not reported in several cases, resulting in biased pre-clinical data [56]. In fact, it has been reported that such studies are 5.3 times more likely to report positive outcomes [57].
A strength in the majority of studies is the use of two different measures to assess cognition, as the use of a land and water maze paradigm has been proposed when reliable data for drug studies needs to be generated [41]. Repetition of behavioral tests in the study of Go et al. [23] for reconfirmation is another way to strengthen the reliability of results. Nevertheless, no long-term follow-up was performed in any papers to assess the effects of treatments after their end period. While this can be explained by the euthanasia of mice for brain analysis, this information will be needed to assess efficacy and safety before clinical trials.
STATISTICAL ANALYSIS AND QUALITY OF REPORTING
Statistical analysis is key in this review as all papers analyzed quantitatively the effects of GM therapies on AD mice. Statistical significance of data was considered for an alpha value of 0.05 and below, consistently across papers. Table 4 details the reported results from the reviewed studies. For two group comparisons, independent two-tailed Student’s t-tests were applied when testing the hypothesis about two means, from the therapy and control groups, based on the assumption of normal distribution [58]. Using t-tests, Abdelhamid et al. [24] reported significant reductions of Aβ production and deposition in hippocampus but not in the cortex of probiotic-treated mice compared to a vehicle group. With the same analytical approach, Kim et al. [22] found significant decrease in Aβ plaque burden in the cerebral cortex and hippocampus after FMT compared to ADLPAPT mice. These opposing results for the cortex may be linked to differential cortical decreases in gliosis; however, the causes are unclear (Table 3). Also, both studies did not perform multiple comparisons with a WT group nor with other doses, which limits the conclusions.
Table 3
Table summarizing results for cognition, neuroinflammation and AD biomarkers (significance is set at alpha value of 0.05)
Treatment Authors | Statistical method | Cognition analysis | Neuroinflammation analysis | Analysis of Aβ plaques and p-tau |
Clostridium butyricum Sun et al. [20] | Statistical differences analyzed by one-way ANOVA followed by Tukey’s test. | Significant remarkable amelioration in MWM and NOR test Data not reported, graph only | Significantly dramatical decrease of microglia activation (levels of CD11b and COX-2) in the cortex. Data not reported, graphs only. | Density of amyloid deposition (Congo red) significantly decreased. Level of Aβ42 significantly decreased. Comparisons with APP/PS1. Data and effect size not reported, graphs only. |
Sodium oligomannate Wang et al. [21] | Statistical tests performed by one-way and two-way ANOVA. | Significant amelioration in MWM and Y maze, compared to APP/PS1 mice, indicated by significantly larger numbers of platform-site crossovers during MWM (F(3, 55) = 6.542) and higher accuracy of the Y maze (F(3, 71) = 12.39). | Significant decrease in microglial activation: measured by positive signal density of Iba1 in hippocampus (F(2, 15) = 21.94), with a concentration of 100 mpk for GV-971. | Significant reduction in Aβ plaque deposition (F(2, 14) = 22.78) and tau phosphorylation (F(2,15) = 13.06), in the hippocampus. |
Fecal transplantation Kim et al. [22] | One-way ANOVA followed by Tukey’s multiple comparison test. Two-tailed unpaired t-test for comparisons of Aβ and tau. | Significant rescue of impairment in Y maze, compared to ADLPAPT mice but no significant differences in the number of total entries. Mixed results in freezing behavior of contextual test and fear-conditioning. Data and effect size not reported, graph only. | Significant decrease in activated microglia (Iba1) and hypertrophic reactive astrocytes (GFAP) in the frontal cortex. Data and effect size not reported, graphs only. | Significant reduction in tau aggregation in the hippocampus. Significant reduction in Aβ plaque burden in the frontal cortex and hippocampus. Both soluble and insoluble Aβ40 levels of the cerebral cortex were significantly decreased. No significant changes for Aβ42 . Data and effect size not reported, graphs only. |
Agathobaculum butyriciproducens Go et al. [23] | One-way ANOVA with post hoc tests. Student’s t-test for comparisons of Aβ and tau. | For APP/PS1 mice, significantly marked increase in discrimination index: Tg-Veh: M = –0.045, SE = 0.03, Tg-SR: M = 0.251, SE = 0.05, p = 0.01 (measured by time); Tg-Veh: M=–0.059, SE = 0.03; Tg-SR: M = 0.21, SE = 0.05, p = 0.01 (measured by number). For LPS mice, significant increase in discrimination index of NOR test: Veh+LPS: M = –0.06, SE = 0.05, SR79 + LPS: M = 0.24, SE = 0.07, p < 0.05 (measured by time); Veh+LPS: M = –0.11, SE = 0.09, SR79 + LPS: M = 0.23, SE = 0.07, p < 0.05 (measured by number). Effect size is not always reported. | In parietal cortex of APP/PS1 mice, microglial activation (Iba1) significantly decreased (Tg-Veh: M = 100.00, SE = 5.18%, Tg-SR: M = 72.92, SE = 4.51%, p = 0.01) and hippocampus (Tg-Veh: M = 100.00, SE = 3.94%, Tg-SR:79.62, SE = 6.03%, p < 0.05) after SR79 treatment. Not significant reduction of astrocytes in parietal cortex (Tg-Veh: M = 100.00, SE = 9.08%, Tg-SR: M = 71.97, SE = 11.04%, p = 0.067). For LPS mice, significant decrease in microglia activation in parietal cortex (Veh+LPS: M = 2.20, SE = 0.25%, SR79 + LPS: M = 1.42, SE = 0.12, p < 0.05) Hippocampus: slightly non-significant decrease (Veh+LPS: M = 4.70, SE = 0.72%, SR79 + LPS: M = 3.55, SE = 0.30, p < 0.05) | Significant decrease in Aβ plaque burden of APP/PS1 mice in the parietal cortex (Tg-Veh: M = 100.00, SE = 8.24%, Tg-SR: M = 72.60, SE = 6.57%, p < 0.05) and hippocampus (Tg-Veh: M = 100.00, SE = 11.89%, Tg-SR: 64.88, SE = 6.26%, p < 0.05). |
Bifidobacterium breve Abdelhamid et al. [24] | Student’s t-test to compare the vehicle group with the probiotic group. | In NOR test, significant increase in exploration time for novel object compared to familiar object. Significantly higher discrimination index in probiotics than vehicle group. Data and effect size not reported, graph in the supplementary material. | Hippocampus: significant decrease in microglial activation (Iba1) Cortex: no significant differences between groups. No significant differences for astrocytes (GFAP) in hippocampus and cortex. Data and effect size not reported, graphs only. | No significant changes in tau phosphorylation in both hippocampus and cortex. Significant reduction in Aβ plaque burden, Aβ levels and fibrils in the hippocampus (both soluble and insoluble). No significance in the cortex. Data and effect size not reported, graphs only. |
BdNlRB Tsering et al. [25] | Two-tailed t-test for comparisons between two groups. One-way ANOVA for comparisons between more than two groups. | Significant improvement in learning ability from MWM after treatment administration (for both Mt and Mi models of AD). For Mt, low and medium doses returned to normal levels (M = 21.98, SD = 23.57 and M = 28.03, SD = 24.37, respectively), high dose was not significant (M = 44.38, SD = 42.98). In Mi mice, results were not significant for the three groups (M = 13.70, SD = 12.963, M = 12.31, SD = 9.69, M = 15.73, SD = 15.05, respectively). No significant improvement in memory ability of Mt mice. | Significant increase of number of microglia (Iba1) by 39.3% and 31.6%, respectively, in the hippocampus. The high dose significantly decreased the number of microglia, compared to APP/PS1 mice. | Significant decrease with low and medium doses of p-tau (Tau) by 8.05% and 12.7%, respectively, in the hippocampus, but no significant decrease in Aβ plaques. The high dose did not produce significant differences from APP/PS1 mice for p-tau and significantly increased Aβ plaques. |
Combination of Anti-Aβ Antibody NP106 and Curcumin Analog TML-6 Su et al. [26] | Two-way and one-way ANOVA with Tukey’s post-hoc tests. | Significant improvement in nesting behavioural test after monotherapy and compared to WT controls too. Combination therapy groups’ performance was higher and statistically comparable to WT controls (numbers and strength are not reported, graph only). Nesting scores were negatively correlated with insoluble Aβ1–42 levels (r = –0.5682, p < 0.001), the percentage of Aβ plaques (r = –0.431, p < 0.01), and the number of Aβ plaques (r = –0.4783, p < 0.01). | Significant increase in percentage of colocalization between microglia (Iba1) and Aβ plaques in combination therapy but not in monotherapy.. Significant morphological changes of microglia in all therapies, with increase in total outgrowth, number of branches and mean process length. No significances for cell body size. Brain slices from hippocampus and cortex. Data and effect size not reported, graphs only | Significant reduction of Aβ plaques (area percentage and number), both for monotherapy and combination therapy (more marked). Among all groups, significant decrease in insoluble forms but not soluble ones of Aβ40 and Aβ42. Data and effect size not reported, graphs only. |
Similarly, Su et al. [26] compared results to AD controls only and identified significant reductions in the number of Aβ plaques after monotherapy and slightly further after combination treatment. For performing such multiple comparisons, analysis of variance (ANOVA) was used here, followed by Tukey’s post hoc tests as ANOVA tests to identify where the difference in comparison lies between conditions. Performing ANOVA, most studies identified significant improvements in learning and memory abilities (p < 0.05). For instance, Wang et al. [21] reported improvements in cognitive impairment in MWM and Y-Maze, stating that GV-971 exhibited significant ameliorative effects in APP/PS1 mice. Results found significantly larger numbers of platform-site crossovers during MWM (F (3, 55) = 6.542) and higher accuracy of the Y-maze (F (3, 71) = 12.39). Sun et al. [20] did not report their results but the histogram for MWM after Clostridium butyricum shows quasi-identical improvements to Wang et al. [21] with the higher dose of 100 mpk of GV-971. Wang et al. and Kim et al. [21, 22] found similar results in the Y-maze testing spatial working memory, as per the graphs. From these studies, it appears that AD mouse models present stronger disturbance of performance in MWM than Y-maze tests. This could suggest that MWM was better suited to assess AD cognitive impairments. However, the frequent lack of data reporting limits comparisons of this review, mostly based on the available histograms without exact numbers.
When stating results, clarity is key for proper understanding and transfer of knowledge to the scientific community. While authors consistently informed on the significance of their results, they did not always state the effect sizes and therefore reporting can often be inconsistent [59]. This is a common limitation that can account for lower reproducibility success [60]. For instance, when stating changes in butyrate levels of APP/PS1 mice compared with CB-treated group, it is only mentioned that they significantly increased [20].
On the other side, when the strength of a variation is included, reporting of results risks being amplified. Indeed, one of the papers qualified the decrease in activated microglia and hypertrophic astrocytes following FMT as dramatical in the frontal cortex [22]. The representative confocal images support a substantial and visible decrease but the graphs quantifying Iba1 and GFAP positive areas support a smaller decrease. This points out the relevance of using several representation formats for the same analysis, allowing both visualization and quantification of results to compare them. In contrast, the experiment conducted by Sun et al. [20] did find significantly high suppression in microglia activation after butyrate treatment compared to controls. The histogram quantifying immunofluorescent intensity of CD11b and western blot analysis showed almost complete reversal of abnormalities. These differences in result sizes could be explained by the treatments themselves or their use of different methods such as study design, immunostaining, or mouse models. Following Agathobaculum butyriciproducens treatment, Go et al. [23] also observed significant decrease of microglial activation while for astrocytes it was non-significant, as indicated by photomicrographs and graphs quantifying percent areas for Iba1.
Inclusion of non-significant differences is important to minimize publication bias and acknowledge honesty in reporting [61]. Additionally, the publication of negative results helps in achieving higher statistical power in research [62]. The study by Tsering et al. [25] illustrates this last point by mentioning that they expected the therapy BdNlRB to remove Aβ protein through metals chelators; however, findings showed that the removal of amyloid plaques was not effective. They then addressed this unpredicted result by hypothesizing that this may be due to the inability for the minerals to cross the BBB.
However, not all papers reflected or provided details on their own limitations [20, 21]. Information is lacking about potential confounding factors, such as sex, or methodological limitations. For example, it is relevant to report whether all animals that started the experiment did complete it because it can cause bias in results [63]. Su et al. [26] reported the spontaneous death of some APP/PS1 mice during the study and performed pathological and behavioral examinations but did not provide further details on the causes nor the impact on findings. Transparency and reflection are especially relevant when potential issues of conflict of interest exist as they may also increase risk of bias. In this review, three papers mention that researchers were employed in biomedical companies developing therapeutics [21, 26]. Another factor potentially impacting research quality is self-citation when comparing results with previous studies [64]. Sun et al. [20] discussed their results highlighting its consistency with their own previous findings such as the increase observed in Helicobacter in APP/PS1 mice. They used the same approach to confirm that SCFA butyrate possesses anti-inflammatory effects (by decreasing proinflammatory cytokines) and neuroprotective effects (by the suppression of microglia activation). While this consistency in findings is positive, further comparison with external studies would increase the potential for generalization and therefore its quality. For instance, Kim et al. [22] argued for community-level alterations of GM rather than single bacterial taxon changes, in line with other studies’ findings.
Finally, there was a lack of information about blinding of researchers who performed the statistical analysis, which could represent a risk of confirmation bias due to researchers being keen to prove their hypothesis [65]. Likewise, only one paper described that power analysis and power calculation of sample size was performed resulting in 6 animals per group [23]. The report affirms that it previously showed this size is sufficient to obtain statistically significant results of parameters analyzed. While it cannot be established whether researchers consulted statisticians for these studies, lack of consultation for the study design, conduct and analysis is a common limitation of animal trials according to Banik et al. [56].
CONCLUSION
To conclude, all recent selected studies provide encouraging findings and validate the microbiota as a new target for the modulation of microglia in both prevention or treatment of AD. Results from in vivo studies confirm the significance of cognitive improvements and prevention along with decrease Aβ burden and neuroinflammation following treatment in mice. Therefore, data from mouse models supports the efficacy of treatments for AD that target the GM. Nevertheless, the effect size remains unclear, and findings are not strong enough to be confident about their translation into clinical settings and the possibility of adoption to the population of interest. The lack of complete reversal of symptoms could mean that additional factors are involved, supporting the multifactorial hypothesis of AD. The consideration of AD as a systemic disease might become a key strategy in treating AD.
Combining the current evidence, the predominant hypothesis starts with the alleviation of the intestinal barrier thanks to the presence of more beneficial GM bacterial communities that regulate gut homeostasis. SCFA butyrate, produced by specific bacteria, is a mediator of the gut-brain axis and its increase appears to have a key role in improving systemic inflammation. Consequently, evidence supports the switching of microglia into neuroprotective actions, with more mRNA expression of anti-inflammatory cytokines and enhancement of Aβ phagocytosis. The decrease in Aβ plaques is demonstrated by all studies. Hippocampal ADAM10 protein increase might allow the inhibition of Aβ production. On a neuronal level, inhibition of neuronal apoptosis and repair of neuronal function may occur, potentially mediated by modulation of protein levels (for example, increase in synaptic proteins).
Combination therapies have the potential to be a new effective treatment approach, perhaps in combination with non-invasive brain stimulation (NIBS) or immunotherapy. The data and rationale are encouraging but it is also more complex to evaluate multiple interactions together. Multitargets treatments should be further explored to clarify in which conditions they present higher efficacy.
For the viability and validation of new treatments with new targets, additional considerations still need to be verified. While the overall rationale of these experiments is robust, human translation is key and yet challenging in the field of AD treatment; more human data is needed in the context of GM treatments. Research combining animal experiments and human data from low-risk settings could become a complementary approach. For safety and efficacy, further experiments are needed to understand why certain thresholds might produce opposite and toxic effects as well as potential contraindications for certain AD patients. Therefore, stratification of patients should be considered in future trials. In addition, the studies assessing probiotics or FMT did not comment on general safety, which will be necessary [66].
Concerning reporting, statistical analysis methods were consistent and minimize the risks of significance errors. However, blinding and randomization from data collection to statistical analysis could be strengthened. Carefully developing statistical analysis plans could increase the quality of findings by determining power analysis, sample size, randomization method, duration of trial and dropout animals’ information [56]. Data was not sufficiently reported to allow precise comparisons, despite the use of graphs and informative subheadings. Few results were negative or non-significant, but their inclusion remains key. Implementing standardized guidelines, such as ARRIVE, could strengthen data reporting in preclinical studies by limiting bias and confounding factors, informing on inclusion and exclusion criteria, co-morbidities, blinding, and missing data [67]. Archiving of secured and retrievable data about the study, materials and facilities has also been recommended to improve data sharing, trace back discrepancies between studies and limit reporting errors [56].
The findings of this critical review present limitations due to the limited number of primary papers appraised. To increase significance and become representative of the current state of research, systematic reviews that add papers from different geographies and different types of GM-related therapies would be valuable. For example, food-based therapies were not analyzed here, despite having been reported as another effective approach [12]. Furthermore, comparing results to standard-of-care treatments for AD would allow to evaluate potential superiority. In the future, building multidisciplinary research groups, at the intersection of neuroscience, immunology, and nutrition will be key to create synergies that will accelerate therapeutical findings for AD.
ACKNOWLEDGMENTS
The authors have no acknowledgments to report.
FUNDING
The authors have no funding to report.
CONFLICT OF INTEREST
The authors have no conflict of interest to report.
SUPPLEMENTARY MATERIAL
[1] The supplementary material is available in the electronic version of this article: https://dx.doi.org/10.3233/ADR-220097.
REFERENCES
[1] | Soria Lopez JA , González HM , Léger GC ((2019) ) Alzheimer’s disease. Handb Clin Neurol 167: , 231–255. |
[2] | Wong W ((2020) ) Economic burden of Alzheimer disease and managed care considerations. Am J Manag Care 26: , S177–S183. |
[3] | Jouanne M , Rault S , Voisin-Chiret AS ((2017) ) Tau protein aggregation in Alzheimer’s disease: An attractive target for the development of novel therapeutic agents. Eur J Med Chem 139: , 153–167. |
[4] | Kodamullil AT , Zekri F , Sood M , Hengerer B , Canard L , McHale D , Hofmann-Apitius M ((2017) ) Trial watch: Tracing investment in drug development for Alzheimer disease. Nat Rev Drug Discov 16: , 819. |
[5] | Schain M , Kreisl WC ((2017) ) Neuroinflammation in neurodegenerative disorders-a review. Curr Neurol Neurosci Rep 17: , 25. |
[6] | Prinz M , Erny D , Hagemeyer N ((2017) ) Ontogeny and homeostasis of CNS myeloid cells. Nat Immunol 18: , 385–392. |
[7] | Czirr E , Wyss-Coray T ((2012) ) The immunology of neurodegeneration. J Clin Invest 122: , 1156–1163. |
[8] | Fakhoury M ((2016) ) Immune-mediated processes in neurodegeneration: Where do we stand? J Neurol 263: , 1683–1701. |
[9] | Imbimbo BP , Watling M ((2021) ) What have we learned from past failures of investigational drugs for Alzheimer’s disease? Expert Opin Investig Drugs 30: , 1175–1182. |
[10] | Abdel-Haq R , Schlachetzki JCM , Glass CK , Mazmanian SK ((2019) ) Microbiome-microglia connections via the gut-brain axis. J Exp Med 216: , 41–59. |
[11] | Erny D , De Angelis ALH , Jaitin D , Wieghofer P , Staszewski O , David E , Keren-Shaul H , Mahlakoiv T , Jakobshagen K , Buch T , Schwierzeck V , Utermöhlen O , Chun E , Garrett WS , Mccoy KD , Diefenbach A , Staeheli P , Stecher B , Amit I , Prinz M ((2015) ) Host microbiota constantly control maturation and function of microglia in the CNS. Nat Neurosci 18: , 965–977. |
[12] | Kowalski K , Mulak A ((2019) ) Brain-gut-microbiota axis in Alzheimer’s disease. J Neurogastroenterol Motil 25: , 48. |
[13] | Roy Sarkar S , Banerjee S ((2019) ) Gut microbiota in neurodegenerative disorders. J Neuroimmunol 328: , 98–104. |
[14] | Bairamian D , Sha S , Rolhion N , Sokol H , Dorothée G , Lemere CA , Krantic S ((2022) ) Microbiota inneuroinflammation and synaptic dysfunction: A focus on Alzheimer’s disease. Mol Neurodegener 17: , 19. |
[15] | Vogt NM , Kerby RL , Dill-McFarland KA , Harding SJ , Merluzzi AP , Johnson SC , Carlsson CM , Asthana S , Zetterberg H , Blennow K , Bendlin BB , Rey FE ((2017) ) Gut microbiome alterations in Alzheimer’s disease. Sci Rep 7: , 13537. |
[16] | Pistollato F , Cano SS , Elio I , Vergara MM , Giampieri F , Battino M ((2016) ) Role of gut microbiota and nutrients in amyloid formation and pathogenesis of Alzheimer disease. Nutr Rev 74: , 624–634. |
[17] | Hoogland ICM , Houbolt C , van Westerloo DJ , van Gool WA , van de Beek D ((2015) ) Systemic inflammation and microglial activation: Systematic review of animal experiments. J Neuroinflammation 12: , 1–13. |
[18] | Figarella K , Wolburg H , Garaschuk O , Duszenko M ((2020) ) Microglia in neuropathology caused by protozoan parasites. Biol Rev 95: , 333–349. |
[19] | Liu L , Huh JR , Shah K ((2022) ) Microbiota and the gut-brain-axis: Implications for new therapeutic design in the CNS. EBioMedicine 77: , 103908. |
[20] | Sun J , Xu J , Yang B , Chen K , Kong Y , Fang N , Gong T , Wang F , Ling Z , Liu J , Sun J , Chen K , Xu J , Yang B , Kong Y , Fang N , Gong T , Liu J ((2019) ) Effect of Clostridium butyricum against microglia-mediated neuroinflammation in Alzheimer’s disease via regulating gut microbiota and metabolites butyrate. Mol Nutr Food Res 64: , 1900636. |
[21] | Wang X , Sun G , Feng T , Zhang J , Huang X , Wang T , Xie Z , Chu X , Yang J , Wang H , Chang S , Gong Y , Ruan L , Zhang G , Yan S , Lian W , Du C , Yang D , Zhang Q , Lin F , Liu J , Zhang H , Ge C , Xiao S , Ding J , Geng M ((2019) ) Sodium oligomannate therapeutically remodels gut microbiota and suppresses gut bacterial amino acids-shaped neuroinflammation to inhibit Alzheimer’s disease progression. Cell Res 29: , 787–803. |
[22] | Kim M-S , Kim Y , Choi H , Kim W , Park S , Lee D , Kim DK , Kim J , Choi H , Hyun D-W , Lee J-Y , Young E , Lee D-S , Bae J-W , Mook-Jung I ((2020) ) Gut microbiota Transfer of a healthy microbiota reduces amyloid and tau pathology in an Alzheimer’s disease animal model. Gut 69: , 283–294. |
[23] | Go J , Chang DH , Ryu YK , Park HY , Lee IB , Noh JR , Hwang DY , Kim BC , Kim KS , Lee CH ((2021) ) Human gut microbiota Agathobaculum butyriciproducens improves cognitive impairment in LPS-induced and APP/PS1 mouse models of Alzheimer’s disease. Nutr Res 86: , 96–108. |
[24] | Abdelhamid M , Zhou C , Ohno K , Kuhara T , Taslima F , Abdullah M , Jung CG , Michikawa M ((2022) ) Probiotic Bifidobacterium breve prevents memory impairment through the reduction of both amyloid-β production and microglia activation in APP knock-in mouse. J Alzheimers Dis 85: , 1555–1571. |
[25] | Tsering J , Chen Q , Li H , Han Y , Wu J , Yin H , Hu J , Su S , Shi X , Hu X , Che B ((2022) ) Effects of the Tibetan medicine Byur dMar Nyer lNga Ril Bu on Alzheimer’s disease in mice models. J Ethnopharmacol 283: , 114724. |
[26] | Su IJ , Hsu CY , Shen S , Chao PK , Hsu JT , Hsueh JT , Liang JJ , Hsu YT , Shie FS ((2022) ) The beneficial effects of combining anti-Aβ antibody NP106 and curcumin analog TML-6 on the treatment of Alzheimer’s disease in APP/PS1 mice. Int J Mol Sci 23: , 556. |
[27] | Roy CC , Kien CL , Bouthillier L , Levy E ((2006) ) Short-chain fatty acids: Ready for prime time? Nutr Clin Pract 21: , 351–366. |
[28] | Stilling RM , van de Wouw M , Clarke G , Stanton C , Dinan TG , Cryan JF ((2016) ) The neuropharmacology of butyrate: The bread and butter of the microbiota-gut-brain axis? Neurochem Int 99: , 110–132. |
[29] | Wenzel TJ , Gates EJ , Ranger AL , Klegeris A ((2020) ) Short-chain fatty acids (SCFAs) alone or in combination regulate select immune functions of microglia-like cells. Mol Cell Neurosci 105: , 103493. |
[30] | Hoyles L , Snelling T , Umlai UK , Nicholson JK , Carding SR , Glen RC , McArthur S ((2018) ) Microbiome-host systems interactions: Protective effects of propionate upon the blood-brain barrier. Microbiome 6: , 55. |
[31] | Holmes C , Cunningham C , Zotova E , Woolford J , Dean C , Kerr S , Culliford D , Perry VH ((2009) ) Systemic inflammation and disease progression in Alzheimer disease. Neurology 73: , 768–774. |
[32] | Morris JK , Honea RA , Vidoni ED , Swerdlow RH , Burns JM ((2014) ) Is Alzheimer’s disease a systemic disease? Biochim Biophys Acta 1842: , 1340–1349. |
[33] | Dodiya HB , Kuntz T , Shaik SM , Baufeld C , Leibowitz J , Zhang X , Gottel N , Zhang X , Butovsky O , Gilbert JA , Sisodia SS ((2019) ) Sex-specific effects of microbiome perturbations on cerebral Ab amyloidosis and microglia phenotypes. J Exp Med 216: , 1542–1560. |
[34] | Cook ND ((2008) ) The neuron-level phenomena underlying cognition and consciousness: Synaptic activity and the action potential. Neuroscience 153: , 556–570. |
[35] | Dai CL , Liu F , Iqbal K , Gong CX ((2022) ) Gut microbiota and immunotherapy for Alzheimer’s disease. Int J Mol Sci 23: , 15230. |
[36] | Mancuso C , Santangelo R ((2018) ) Review Alzheimer’s disease and gut microbiota modifications: The long way between preclinical studies and clinical evidence. Pharmacol Res 129: , 329–336. |
[37] | Nguyen TLA , Vieira-Silva S , Liston A , Raes J ((2015) ) How informative is the mouse for human gut microbiota research? Dis Model Mech 8: , 1–16. |
[38] | Fisher EMC , Bannerman DM ((2019) ) Mouse models of neurodegeneration: Know your question, know your mouse. Sci Transl Med 11: , 1818. |
[39] | Jensen MN , Ritskes-Hoitinga M ((2007) ) How isoflavone levels in common rodent diets can interfere with the value of animal models and with experimental results. Lab Anim 41: , 1–18. |
[40] | Savonenko A , Xu GM , Melnikova T , Morton JL , Gonzales V , Wong MPF , Price DL , Tang F , Markowska AL , Borchelt DR ((2005) ) Episodic-like memory deficits in the APPswe/PS1dE9 mouse model of Alzheimer’s disease: Relationships to beta-amyloid deposition and neurotransmitter abnormalities. Neurobiol Dis 18: , 602–617. |
[41] | Sharma S , Rakoczy S , Brown-Borg H ((2010) ) Assessment of spatial memory in mice. Life Sci 87: , 521–536. |
[42] | Zahs KR , Ashe KH ((2010) ) ‘Too much good news’ – are Alzheimer mouse models trying to tell us how to prevent, not cure, Alzheimer’s disease? Trends Neurosci 33: , 381–389. |
[43] | Pippin JJ , Cavanaugh SE , Pistollato F ((2019) ) Animal research for Alzheimer disease: Failures of science and ethics. In Brill, pp. Animal Experimentation: Working Towards a Paradigm Change480–516. |
[44] | Festing MFW , Altman DG ((2002) ) Guidelines for the design and statistical analysis of experiments using laboratory animals. ILAR J 43: , 244–258. |
[45] | Richter SH , Garner JP , Würbel H ((2009) ) Environmental standardization: Cure or cause of poor reproducibility in animal experiments? Nat Methods 6: , 257–261. |
[46] | Cheng LH , Liu YW , Wu CC , Wang S , Tsai YC ((2019) ) Psychobiotics in mental health, neurodegenerative and neurodevelopmental disorders. J Food Drug Anal 27: , 632–648. |
[47] | Nair A , Morsy MA , Jacob S ((2018) ) Dose translation between laboratory animals and human in preclinical and clinical phases of drug development. Drug Dev Res 79: , 373–382. |
[48] | Zhu G , Zhao J , Zhang H , Chen W , Wang G , Bautista-Gallego J ((2021) ) Probiotics for mild cognitive impairment and Alzheimer’s disease: A systematic review and meta-analysis. Foods 10: , 1672. |
[49] | Ouwehand AC ((2017) ) A review of dose-responses of probiotics in human studies. Benef Microbes 8: , 143–151. |
[50] | Shin J , Lee S , Go MJ , Lee SY , Kim SC , Lee CH , Cho BK ((2016) ) Analysis of the mouse gut microbiome using full-length 16S rRNA amplicon sequencing. Sci Rep 6: , 29681. |
[51] | Peng W , Yi P , Yang J , Xu P , Wang Y , Zhang Z , Huang S , Wang Z , Zhang C ((2018) ) Association of gut microbiota composition and function with a senescence-accelerated mouse model of Alzheimer’s Disease using 16S rRNA gene and metagenomic sequencing analysis. Aging (Albany NY) 10: , 4054. |
[52] | Ramos-Vara JA ((2005) ) Technical aspects of immunohistochemistry. Vet Pathol 42: , 405–426. |
[53] | Menter T , Bachmann M , Grieshaber S , Tzankov A ((2017) ) A more accurate approach to amyloid detection and subtyping: Combining in situ Congo red staining and immunohistochemistry. Pathobiology 84: , 49–55. |
[54] | Mocellin S , Provenzano M , Rossi CR , Pilati P , Nitti D , Lise M ((2003) ) Use of quantitative real-time PCR to determine immune cell density and cytokine gene profile in the tumor microenvironment. J Immunol Methods 280: , 1–11. |
[55] | Vorhees C V. , Williams MT ((2006) ) Morris water maze: Procedures for assessing spatial and related forms of learning and memory. Nat Protoc 1: , 848–858. |
[56] | Banik A , Brown RE , Bamburg J , Lahiri DK , Khurana D , Friedland RP , Chen W , Ding Y , Mudher A , Padjen AL , Mukaetova-Ladinska E , Ihara M , Srivastava S , Srivastava MVP , Masters CL , Kalaria RN , Anand A ((2015) ) Translation of pre-clinical studies into successful clinical trials forAlzheimer’s disease: What are the roadblocks and how can they be overcome? J Alzheimers Dis 47: , 815–843. |
[57] | Kilkenny C , Parsons N , Kadyszewski E , Festing MFW , Cuthill IC , Fry D , Hutton J , Altman DG ((2009) ) Survey of the quality of experimental design, statistical analysis and reporting of research using animals. PLoS One 4: , e7824. |
[58] | Kim TK ((2015) ) T test as a parametric statistic. Korean J Anesthesiol 68: , 540–546. |
[59] | Bakker M , Wicherts JM ((2011) ) The (mis)reporting of statistical results in psychology journals. Behav Res Methods 43: , 666–678. |
[60] | Festing MFW ((2006) ) Design and statistical methods in studies using animal models of development. ILAR J 47: , 5–14. |
[61] | ter Riet G , Korevaar DA , Leenaars M , Sterk PJ , van Noorden CJF , Bouter LM , Lutter R , Elferink RPO , Hooft L ((2012) ) Publication bias in laboratory animal research: A survey on magnitude, drivers, consequences and potential solutions. PLoS One 7: , e43404. |
[62] | Nosek BA , Lakens D ((2014) ) Registered reports: A method to increase the credibility of published results. Soc Psychol 45: , 137. |
[63] | Landis SC , Amara SG , Asadullah K , Austin CP , Blumenstein R , Bradley EW , Crystal RG , Darnell RB , Ferrante RJ , Fillit H , Finkelstein R , Fisher M , Gendelman HE , Golub RM , Goudreau JL , Gross RA , Gubitz AK , Hesterlee SE , Howells DW , Huguenard J , Kelner K , Koroshetz W , Krainc D , Lazic SE , Levine MS , Macleod MR , Mccall JM , Moxley Iii RT , Narasimhan K , Noble LJ , Perrin S , Porter JD , Steward O , Unger E , Utz U , Silberberg SD ((2012) ) A call for transparent reporting to optimize the predictive value of preclinical research. Nature 490: , 187–191. |
[64] | Penders B ((2018) ) Ten simple rules for responsible referencing. PLoS Comput Biol 14: , e1006036. |
[65] | MacCoun RJ , Perlmutter S ((2017) ) Blind analysis as a correction for confirmatory bias in physics and in psychology. In Psychological Science Under Scrutiny: Recent Challenges and Proposed Solutions, First Edition, Lilienfeld SO, Waldman ID, eds. John Wiley & Sons, Inc., pp. 295–322. |
[66] | Anadón A , Ares I , Martínez-Larrañaga MR , Martínez MA ((2021) ) Probiotics: Safety and toxicity considerations. In Nutraceuticals (Second Edition), Gupta RC, Lall R, Srivastava A, eds. Academic Press pp. 1081–1105. |
[67] | Kilkenny C , Browne WJ , Cuthill IC , Emerson M , Altman DG ((2010) ) Improving bioscience research reporting: The ARRIVE guidelines for reporting animal research. J Pharmacol Pharmacother 1: , 94–99. |