Induction of Gut Microbial Tryptamine by SARS-CoV-2 in Nonhuman Primate Model Consistent with Tryptamine-Induced Model of Neurodegeneration
Abstract
The author discussed recently the possible molecular mechanisms that cause the COVID-19 disease symptoms. Here the analysis of the recent experimental data supports the hypothesis that production of the gut microbial tryptamine can be induced by the SARS-CoV-2 fecal viral activity due to the selective pressure or positive selection of tryptamine-producing microorganisms. In this report, the author suggests that the mechanism of microbial selection bases on the abilities of tryptamine to affect the viral nucleic acid. In other words, the gut microorganisms producing tryptamine are more resistant to SARS-CoV-2 fecal viral activity than microorganisms producing no tryptamine. Earlier we demonstrated the induction of neurodegeneration by tryptamine in human cells and mouse brain. Furthermore, we were able to uncover the human gut bacteria associated with Alzheimer’s disease (AD) using PCR testing of human fecal samples with the new-designed primers targeting the tryptophan-tryptamine pathway. Likely, SARS-CoV-2 is one of the selective pressure factors in the cascade accelerating the neurodegenerative process in AD. This suggestion is consistent with a higher proportion of AD patients among COVID-19 related victims. Gut microbial tryptamine increase due to the viral infection-induced dysbiosis can synergize and potentiate the tryptamine cytotoxicity, necrotizing ability and other properties as a virulence factor.
INTRODUCTION
The health authorities reported that 3,019 Chinese health workers were infected with the severe acute respiratory syndrome coronavirus 2 (SARS-CoV-2), of whom, ten died [1] or 0.33%. No data on preexisting medical conditions in the health workers were provided in this article. However, in recent years, Chinese health workers are often confronted with frustrating situations in the health care system including serious workplace violence against clinicians. A meta-analysis found that the overall prevalence of workplace violence was 62.4% among Chinese health workers [1, 2]. The frequency and mortality of COVID-19 in patients with Alzheimer’s disease (AD) and frontotemporal dementia (FTD) were evaluated in Spain [3]. An observational case series was analyzed. 204 patients were enrolled, 15.2% of whom were diagnosed with COVID-19, and 41.9% of patients with the infection died. COVID-19 occurred in 7.3% of patients living at home, but 72.0% of those living at care homes. Living in care facilities and diagnosis of AD were independently associated with a higher probability of death. The authors found that living in care homes is the most relevant factor for an increased risk of COVID-19 infection and death, with AD patients exhibiting a higher risk than those with FTD [3].
Recently I discussed the possible molecular mechanisms underlying the COVID-19 disease symptoms [4, 5]. We demonstrated the induction of neurodegeneration by biogenic amine tryptamine in human cells and mouse brain [6]. Furthermore, we were able to discover the human gut bacteria associated with Alzheimer’s disease (AD) using PCR testing of human fecal samples with the new-designed primers targeting the tryptophan-tryptamine pathway [7]. The underlying mechanisms of neurotoxic activity of tryptamine are the inhibition of protein biosynthesis enzyme tryptophany-tRNA synthetase (TrpRS) and cholinesterases [4, 8]. The role of TrpRS in neurodegeneration is corroborated by the data on TrpRS mutations in humans. Tryptophanyl tRNA synthetase gene mutations have been associated with multiple neurological phenotypes as reviewed previously [8]. A new case of a severe form of infantile-onset Parkinsonism was recently characterized functionally with biallelic variants in WARS2, encoding the mitochondrial tryptophanyl tRNA synthetase (mtTrpRS) [9]. A substantial reduction of mtTrpRS levels in mitochondria and reduced OXPHOS function was demonstrated, supporting their pathogenicity [9]. Both TrpRS gene mutations and TrpRS inhibition by tryptamine diminish the TrpRS enzymatic activity thus interfering with tRNA aminoacylation, the first vital step of protein biosynthesis [4, 8].
Furthermore, tryptamine is an inhibitor of cholinesterases. Tryptamine is more potent inhibitor of the cholinesterases in histopathologic structures plaques and tangles of AD patients than of those in normal axons and cell bodies [10].
The biogenic amines polyamines produced by gut microbiome alter DNA structure by facilitating the conformational transition from the B form to the Z form or by bending DNA. Furthermore, up to 80% of polyamines in the cell are directly associated with RNA, and spermine has also been implicated in the stabilization of tRNA structure [11]. Polyamines have now been suggested to have a role in the replication of viruses across all known viral replication strategies and most viral families [11]. Brain polyamine levels are altered in AD. As compared with the controls, mean levels of spermidine were markedly and significantly increased (70%) whereas putrescine levels were decreased (28%) in temporal cortex of the AD patients [12]. Tryptamine was shown to be a good competitive inhibitor of diamine oxidase [13], an enzyme catalyzing degradation of diamines and polyamines including histamine, putrescine and spermidine. Therefore, the role of tryptamine in the virus infection is suggested here.
GUT MICROBIOTA, COVID-19, DEMENTIA, AND BIOGENIC AMINES
Zuo et al. reported in 2021 the SARS-CoV-2 fecal viral activity in association with gut microbiota composition in patients with COVID-19 [14]. All patients with COVID-19 were admitted to the Prince of Wales Hospital or the United Christian Hospital, Hong Kong. Fecal viral metagenome of three patients continued to display active viral infection signature up to 6 days after clearance of SARS-CoV-2 from respiratory samples. Faecal samples with signature of high SARS-CoV-2 infectivity had higher abundances of bacterial species Collinsella aerofaciens, Collinsella tanakaei, Streptococcus infantis, and Morganella morganii [14]. Authors of this study suggest the passage or transmission of the extraintestinal microbes into the gut in COVID-19 setting [14].
Elevated levels of histamine, tyramine, cadaverine, and tryptamine in tuna inoculated with M. morganii or Proteus mirabilis were reported by Ahmed in 2019 [15]. Tryptamine formation in canned tuna fish was stimulated (up to 5.4-fold) by inoculation with M. morganii or Proteus mirabilis [15].
Morganella morganii (in the Proteobacteria phylum) has been identified as a causative agent of opportunistic infections and histamine poisoning [16]. M. morganii, a motile gram-negative rod belonging to the family Enterobacteriaceae, has low pathogenicity, but compromised patients can develop diarrhea, wound infections, urinary tract infections, bacteremia, and sepsis due to M. morganii. In addition, M. morganii is known as a major histamine producer due to its powerful histidine decarboxylase. M. morganii is responsible for histamine accumulation in food, which often causes histamine poisoning (scombroid poisoning) in decomposed fishery products. M. morganii was detected in the gill and skin of mackerel, sardine, and albacore. Although many cases present with mild symptoms, rare cases present with severe symptoms that can be potentially life-threatening [16].
Accumulated data have demonstrated that M. morganii can cause various infections, such as sepsis, abscess, purple urine bag syndrome (PUBS), chorioamnionitis, and cellulitis. This bacterium often results in a high mortality rate in patients with some infections [17].
Biochemical and bacteriological investigation of six cases of PUBS was conducted in a geriatric ward for dementia [18]. Muneoka et al. investigated 6 cases exhibiting PUBS (3 males and 3 females). All cases had chronic constipation. Oral ingestion was impossible in one case. PUBS disappeared after antibiotic treatment (3 cases) or spontaneously (one case). In bacterial culture of urine during the exhibition of PUBS, Enterococcus faecalis was isolated together with M. morganii (3 cases) and Pseudomonas aeruginosa (one case). Single infections by Klebsiella pneumoniae or Citrobacter species were also found [18].
Sokol et al. reported in 2021 [19] the usage of a nonhuman primate model (the macaque), that recapitulates mild COVID-19 symptoms, to analyze the effects of a SARS-CoV-2 infection on dynamic changes of the gut microbiota. SARS-CoV-2 infection in a nonhuman primate is associated with changes in the gut microbiota’s composition and functional activity [19]. An overall increase in the amount of indoles was observed, although this signal was mainly driven by increased amounts of 3-indole sulfate and tryptamine. The authors found that the concentrations of metabolites from the serotonin pathway increased in parallel with that of tryptamine, and that there was a strong correlation between the abundances of tryptamine (up to ∼150 nmol/g) and serotonin (∼4 nmol/g) in the targeted fecal metabolomics [19]. 16S rRNA gene profiling and analysis of β diversity indicated significant changes in the composition of the gut microbiota with a peak at 10–13 days post-infection (dpi) with SARS-CoV-2 infection in a nonhuman primate. Analysis of bacterial abundance correlation networks confirmed disruption of the bacterial community at 10–13 dpi. Some alterations in microbiota persisted after the resolution of the infection until day 26. Some changes in the relative bacterial taxon abundance associated with infectious parameters. Interestingly, the relative abundance of Acinetobacter (Proteobacteria) and some genera of the Ruminococcaceae family (Firmicutes) was positively correlated with the presence of SARS-CoV-2 in the upper respiratory tract [19]. Acinetobacter lowffi have been reported as histamine formers [20].
Amato et al. compared gut microbiome composition and functional potential in 14 populations of humans from ten nations and 18 species of wild, non-human primates [21]. In this study, the human gut microbiome composition and functional potential are more similar to those of cercopithecines, a subfamily of Old World monkey, particularly baboons, than to those of African apes. Additionally, the data reveal more inter-individual variation in gut microbiome functional potential within the human species than across other primate species, suggesting that the human gut microbiome may exhibit more plasticity in response to environmental variation compared to that of other primates. Overall, these findings show that diet, ecology, and physiological adaptations are more important than host-microbe co-diversification in shaping the human microbiome [21].
The human gut microbiome is comprised of densely colonizing microorganisms including bacteriophages (phages), the viruses that infect bacteria. In the study by Hsu et al. (2019), gnotobiotic mice were colonized with defined bacteria and subjected to predation by cognate lytic phages [22]. Researchers found that phage predation not only directly impacts susceptible bacteria but also leads to cascading effects on other bacterial species via interbacterial interactions. Metabolomic profiling revealed that shifts in the microbiome caused by phage predation have a direct consequence on the gut metabolome. In some cases, the specificity of phage predation allows for the targeting of bacterial species and consequently the knockdown of uniquely associated metabolic products. Tryptamine is produced via tryptophan decarboxylation by a small number of commensal gut bacteria. During treatment with the phages, the authors detected a 10-, 17-, and 2-fold reduction in tryptamine (0.3, 2, and 13 days, respectively). This corresponds with an 840-, 4-, and 4-fold reduction in Clostridium sporogenes, respectively [22]. Data on protein homologs of tryptophan decarboxylases that produce tryptamine in the defined consortia bacterial strains are provided in this article [22]: Bacteroides fragilis, Clostridium sporogenes, Enterococcus faecalis, Escherichia coli, Akkermansia muciniphila, Bacteroides ovatus, Bacteroides vulgatus, Klebsiella oxytoca, Parabacteroides distasonis, Proteus mirabilis, Ruminococcus gnavus. BLAST search of tryptophan decarboxylase amino acid sequences from R. gnavus (rumgna_01526) and C. sporogenes (clospo_02083) against the other members of bacterial consortium showed poor protein homology (top hit of 31% identity) [22], consistent with my reported data [4, 8].
Therefore, opportunistic pathogen M. morganii that can cause various infections was found in association with COVID-19 and dementia in patients. M. morganii produce biogenic amines and particularly tryptamine. Furthermore, gut microbial tryptamine enrichment is associated with SARS-CoV-2 infection of nonhuman primate [19]. Taken together the data support the SARS-CoV-2 viral infection-induced gut dysbiosis. Direct and indirect interactions have been observed between bacteria and viruses [23, 24]. Effects of viruses on bacterial functions under contrasting nutritional conditions were investigated for four species of bacteria isolated from Hong Kong waters [25]. In this study, bacterial species belonging to Proteobacteria showed differences in resisting viral attack, which can influence the dominance and biodiversity of bacterial species in coastal waters [25]. The results strongly indicate that the lysed bacterial cells were used to support the growth and respiration of the remaining bacterial cells [25].
It can be hypothesized here that tryptamine may protect tryptamine-producing bacterial cells against attack by SARS-CoV-2 and some other viruses due to 1) the tryptamine ability to inhibit the virus assembly, 2) tryptamine binding to the viral DNA or RNA, and 3) tryptamine inhibition of biosynthesis of the viral proteins in the bacterial host cells. It was demonstrated earlier that the assembly of infective particles of bacteriophages lambda and phi80 from heads and tails was inhibited by L-tryptophan and some of its analogues, most notably tryptamine. [26]. Both the rate of assembly and the final yield of phage were inhibited. Results suggest that inhibition is due to interaction of inhibitors with the head substructure [26]. The study by Helene et al. demonstrated the tryptamine binding to nucleic acids [27]. The stacking interaction of tryptamine occurs not only with native DNA but also with denatured DNA and single stranded polynucleotides [27]. Furthermore the results suggest the tryptamine pathway-mediated DNA fragmentation in plant [28], mammalian and human [4] cells. Tryptamine is a very strong basic compound based on its pKa value 10.2 (https://foodb.ca/compounds/FDB000917).
Being obligate intracellular parasites, viruses heavily depend on host cell structures and functions to complete their life cycle, and they also use the translational apparatus of the infected cell to express their proteins [29]. Thus, tryptamine, an inhibitor of bacterial tryptophanyl RNA synthetase can interfere with the biosynthesis of viral proteins in the tryptamine-producing bacteria. Furthermore, tryptamine derivatives demonstrated anti-hepatitis B virus (HBV) activity and cytotoxicity in the HepG2.2.15 cell line [30]. Cytotoxicity and antiviral activity was demonstrated for tryptamine derivative 8 against HIV-1 and its mutants [31].
CONCLUSIONS AND REMARKS
In this report, the author discusses the role of gut microbiome and biogenic amines in the SARS-CoV-2 infection and COVID-19 disease. Tryptamine can affect the viral nucleic acids directly via binding or indirectly via alterations in the levels of diamines and polyamines (Fig. 1). Gut microbial tryptamine increase due to the viral infection-induced dysbiosis can synergize and potentiate the tryptamine cytotoxicity and other abilities attributed to tryptamine as a virulence factor [4].
Fig. 1
SARS-CoV-2 induces dysbiosis in gut microbiome of nonhuman primates thus resulting in tryptamine overproduction.
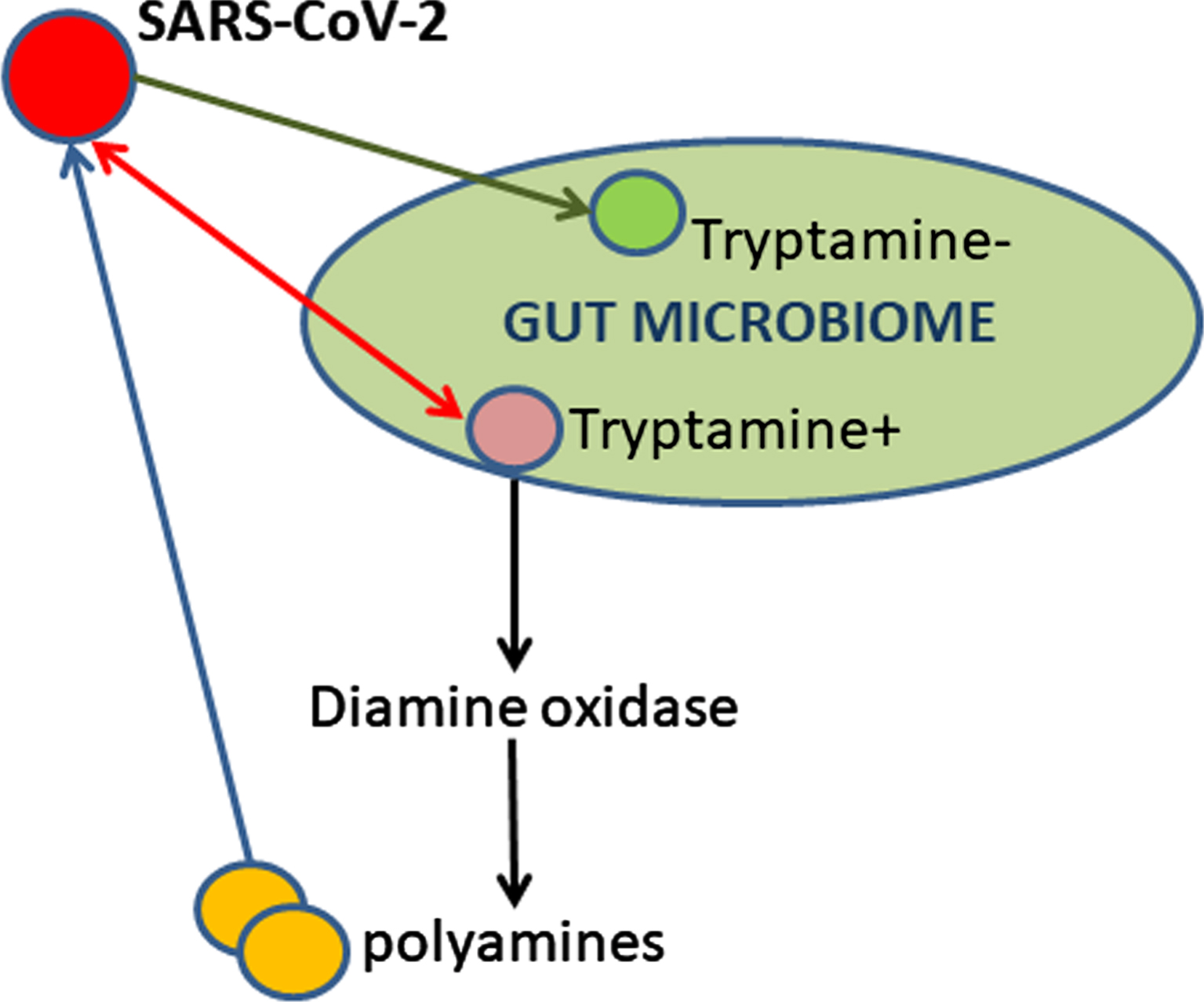
Price reported in 1978 [32] that the increased tryptaminuria which follows alcohol ingestion can be abolished by the concurrent ingestion of alkali even when the amount of alkali consumed is insufficient to increase urinary pH to 6.5 (above which pH a fall in urinary tryptamine may be anticipated, from previous studies, to occur).
Of note, Gardini et al. reported in 2006 [33] that in the method for detection of biogenic amines in bacteria proposed by Bover-Cid and Holzapfel (1999) [34], almost all the controls were positive, probably due to a too rich medium, specifically due to the presence of peptone [33].
CONFLICT OF INTEREST
The author has no conflict of interest to report.
REFERENCES
[1] | Xiang YT , Jin Y , Wang Y , Zhang Q , Zhang L , Cheung T ((2020) ) Tribute to health workers in China: A group of respectable population during the outbreak of the COVID-19. Int J Biol Sci 16: , 1739–1740. |
[2] | Lu L , Dong M , Wang SB , Zhang L , Ng CH , Ungvari GS , Li J , Xiang YT ((2020) ) Prevalence of workplace violence against health-care professionals in China: A comprehensive meta-analysis of observational surveys. Trauma Violence Abuse 21: , 498–509. |
[3] | Matias-Guiu JA , Pytel V , Matias-Guiu J ((2020) ) Death rate due to COVID-19 in Alzheimer’s disease and frontotemporal dementia. J Alzheimers Dis 78: , 537–541. |
[4] | Paley EL ((2021) ) Microbial Metabolism and Disease, Elsevier Science/Academic Press Book. |
[5] | Paley EL ((2021) ) Towards understanding COVID-19: Molecular insights, co-infections, associated disorders, and aging. J Alzheimers Dis Rep 5: , 571–600. |
[6] | Paley EL , Denisova G , Sokolova O , Posternak N , Wang X , Brownell AL ((2007) ) Tryptamine induces tryptophanyl-tRNA synthetase-mediated neurodegeneration with neurofibrillary tangles in human cell and mouse models. Neuromolecular Med 9: , 55–82. |
[7] | Paley EL , Merkulova-Rainon T , Faynboym A , Shestopalov VI , Aksenoff I ((2018) ) Geographical distribution and diversity of gut microbial NADH:ubiquinone oxidoreductase sequence associated with Alzheimer’s disease. J Alzheimers Dis 61: , 1531–1540. |
[8] | Paley EL ((2020) ) Protein Biosynthesis Interference in Disease. Academic Press Book - Elsevier. |
[9] | Martinelli S , Cordeddu V , Galosi S , Lanzo A , Palma E , Pannone L , Ciolfi A , Di Nottia M , Rizza T , Bocchinfuso G , Traversa A , Caputo V , Farrotti A , Carducci C , Bernardini L , Cogo S , Paglione M , Venditti M , Bentivoglio A , Ng J , Kurian MA , Civiero L , Greggio E , Stella L , Trettel F , Sciaccaluga M , Roseti C , Carrozzo R , Fucile S , Limatola C , Di Schiavi E , Tartaglia M , Leuzzi V ((2020) ) Co-occurring WARS2 and CHRNA6 mutations in a child with a severe form of infantile parkinsonism. Parkinsonism Relat Disord 72: , 75–79. |
[10] | Wright CI , Guela C , Mesulam MM ((1993) ) Protease inhibitors and indoleamines selectively inhibit cholinesterases in the histopathologic structures of Alzheimer disease. Proc Natl Acad Sci U S A 90: , 683–686. |
[11] | Mounce BC , Olsen ME , Vignuzzi M , Connor JH ((2017) ) Polyamines and their role in virus infection. Microbiol Mol Biol Rev 81: , e00029–17. |
[12] | Morrison LD , Kish SJ ((1995) ) Brain polyamine levels are altered in Alzheimer’s disease. Neurosci Lett 197: , 5–8. |
[13] | Finazzi-Agro A , Floris G , Fadda MB , Crifo C ((1979) ) Inhibition of diamine oxidase by antihistaminic agents and related drugs. Agents Actions 9: , 244–247. |
[14] | Zuo T , Liu Q , Zhang F , Lui GC , Tso EY , Yeoh YK , Chen Z , Boon SS , Chan FK , Chan PK , Ng SC ((2021) ) Depicting SARS-CoV-2 faecal viral activity in association with gut microbiota composition in patients with COVID-19. Gut 70: , 276–284. |
[15] | Ahmed OM ((2019) ) Histamine and other biogenic amines formation in canned tuna fish inoculated with Morganella morganii or proteus mirabilis in determining food safety during temperature abuse. Nutr Food Toxicol 3: , 690–700. |
[16] | Yamaki S , Omachi T , Kawai Y , Yamazaki K ((2014) ) Characterization of a novel Morganella morganii bacteriophage FSP1 isolated from river water. FEMS Microbiol Lett 359: , 166–172. |
[17] | Liu H , Zhu J , Hu Q , Rao X ((2016) ) Morganella morganii, a non-negligent opportunistic pathogen. Int J Infect Dis 50: , 10–17. |
[18] | Muneoka K , Igawa M , Kurihara N , Kida J , Mikami T , Ishihara I , Uchida J , Shioya K , Uchida S , Hirasawa H ((2008) ) [Biochemical and bacteriological investigation of six cases of purple urine bag syndrome (PUBS) in a geriatric ward for dementia]. Nihon Ronen Igakkai Zasshi 45: , 511–519. |
[19] | Sokol H , Contreras V , Maisonnasse P , Desmons A , Delache B , Sencio V , Machelart A , Brisebarre A , Humbert L , Deryuter L , Gauliard E , Heumel S , Rainteau D , Dereuddre-Bosquet N , Menu E , Ho Tsong Fang R , Lamaziere A , Brot L , Wahl C , Oeuvray C , Rolhion N , Van Der Werf S , Ferreira S , Le Grand R , Trottein F ((2021) ) SARS-CoV-2 infection in nonhuman primates alters the composition and functional activity of the gut microbiota. Gut Microbes 13: , 1–19. |
[20] | Visciano P , Schirone M , Tofalo R , Suzzi G ((2012) ) Biogenic amines in raw and processed seafood. Front Microbiol 3: , 188. |
[21] | Amato KR , Mallott EK , McDonald D , Dominy NJ , Goldberg T , Lambert JE , Swedell L , Metcalf JL , Gomez A , Britton GAO , Stumpf RM , Leigh SR , Knight R ((2019) ) Convergence of human and Old World monkey gut microbiomes demonstrates the importance of human ecology over phylogeny. Genome Biol 20: , 201. |
[22] | Hsu BB , Gibson TE , Yeliseyev V , Liu Q , Lyon L , Bry L , Silver PA , Gerber GK ((2019) ) Dynamic modulation of the gut microbiota and metabolome by bacteriophages in a mouse model. Cell Host Microbe 25: , 803–814 e805. |
[23] | Neu U , Mainou BA ((2020) ) Virus interactions with bacteria: Partners in the infectious dance. PLoS Pathog 16: , e1008234. |
[24] | Almand EA , Moore MD , Jaykus LA ((2017) ) Virus-bacteria interactions: An emerging topic in human infection. Viruses 9: , 58. |
[25] | Liu H , Yuan X , Xu J , Harrison PJ , He L , Yin K ((2015) ) Effects of viruses on bacterial functions under contrasting nutritional conditions for four species of bacteria isolated from Hong Kong waters. Sci Rep 5: , 14217. |
[26] | Deeb SS ((1973) ) Involvement of a tryptophan residue in the assembly of bacteriophages 80 and lambda. J Virol 11: , 353–358. |
[27] | Helene C , Dimicoli JL , Brun F ((1971) ) Binding of tryptamine and 5-hydroxytryptamine (serotonin) to nucleic acids. Fluorescence and proton magnetic resonance studies. Biochemistry 10: , 3802–3809. |
[28] | Ueno M , Imaoka A , Kihara J , Arase S ((2008) ) Tryptamine pathway-mediated DNA fragmentation is involved in sekiguchi lesion formation for light-enhanced resistance in lesion mimic mutant of rice to Magnaporthe grisea infection. J Phytopathol 156: , 715–724. |
[29] | Nakagawa K , Lokugamage KG , Makino S ((2016) ) Viral and cellular mRNA translation in coronavirus-infected cells. Adv Virus Res 96: , 165–192. |
[30] | Qu SJ , Wang GF , Duan WH , Yao SY , Zuo JP , Tan CH , Zhu DY ((2011) ) Tryptamine derivatives as novel non-nucleosidic inhibitors against hepatitis B virus. Bioorg Med Chem 19: , 3120–3127. |
[31] | Sanna G , Madeddu S , Giliberti G , Piras S , Struga M , Wrzosek M , Kubiak-Tomaszewska G , Koziol AE , Savchenko O , Lis T , Stefanska J , Tomaszewski P , Skrzycki M , Szulczyk D ((2018) ) Synthesis and biological evaluation of novel indole-derived thioureas. Molecules 23: , 2554. |
[32] | Price J ((1978) ) The mechanism underlying increased tryptaminuria after alcohol ingestion. Clin Chim Acta 83: , 61–65. |
[33] | Gardini F , Tofalo R , Belletti N , Iucci L , Suzzi G , Torriani S , Guerzoni ME , Lanciotti R ((2006) ) Characterization of yeasts involved in the ripening of Pecorino Crotonese cheese. Food Microbiol 23: , 641–648. |
[34] | Bover-Cid S , Holzapfel WH ((1999) ) Improved screening procedure for biogenic amine production by lactic acid bacteria. Int J Food Microbiol 53: , 33–41. |